GM Technology – Risky Method or Valuable Tool?
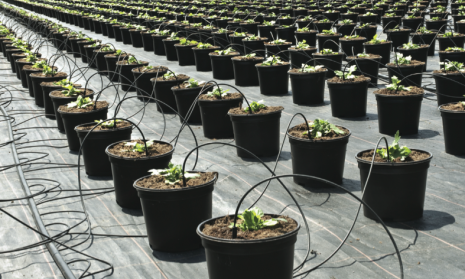
Summary
Genetic modification is one of many current tools that can be used in the development of new, improved crops.
It is widely recognised that in order to meet future requirements for food, without having serious impacts on the environment, and while responding to climate change, we will need to draw on all available technologies.
However, a key question is whether the GM technology itself has inherent risks associated with it, or whether it is essentially benign.
Like any other technology, genetic modification could be used wisely or unwisely. Here, specific applications are not considered, but the actual technology is examined, identifying the similarities and differences between genetic modification and other breeding techniques.
By breaking down the technology into its component parts, it is possible to find relevant comparisons from within the range of conventional breeding technologies or from natural processes which inform the assessment of risks linked to GM methods.
The key components of the GM process including the tissue culture steps, the introduction of DNA, the selection of GM material and features of the introduced DNA are considered.
By making the relevant comparisons to existing conventional techniques and to natural processes, drawing on increasing knowledge of the characteristics of plant genomes, and from the vast literature looking at GM safety, it is possible to assess some of the main concerns linked to GM technology.
The conclusion reached is that the technology itself poses no greater risks than those posed using conventional breeding techniques.
In addition, many past concerns can now be effectively eliminated by developments in GM technology that allow precise changes to be made to plant genomes without the necessity of including additional genes or sequences.
Keywords: Genetic modification, plant breeding, mutation breeding, tissue culture, particle bombard- ment, Agrobacterium, genome engineering
Glossary of terms
Agrobacterium tumefaciens is a soil bacterium that causes crown gall disease in many plant species. The disease symptoms are caused by the transfer of DNA (T-DNA) from the bacterium to the plant. Agrobacterium is now widely used for plant genetic modification.
Backbone sequence is the part of the transformation vector outside of the T-DNA.
Callus is a disorganised mass of plant cells that can sometimes be induced to form organised structures and regenerate plants.
Genetic modification is often referred to as genetic engineering or transformation and is a technology that allows changes/additions to be made to the DNA of a plant or other organism to give it a new and useful characteristic, theoretically using DNA from any biological source.
Genome refers to the entire genetic material, usually DNA, of a plant or other organism.
Genome engineering refers to a range of technologies that allow specific changes to be made to an organism’s DNA at precise locations within the genome.
Horizontal gene transfer is the transfer of DNA between species without the use of reproduction or human intervention.
Hybridisation is the process of crossing two different, but closely related plants to produce a hybrid containing characteristics from both parents.
Mutation breeding is a plant breeding tool where chemicals or radiation are used to create mutations in a plant’s DNA to create useful variation.
Particle gun sometimes referred to as the gene gun is a device that delivers DNA coated microscopic gold particles into plants cells.
Promoter regions are located upstream of a gene and control the expression of the gene.
RNAi is RNA interference, a natural process that is able to reduce the activity of genes or silence them; it is sometimes referred to as RNA-induced gene silencing.
Somaclonal variation is the variation caused by the passage of plant material through tissue culture; this usually involves a disorganised callus phase.
TALENs (Transcription activator-like effector nucleases) consist of a fusion between a DNA binding domain and a nuclease such as Fok1. They can be engineered to bind specific DNA sequences and used to create changes at specific locations in the genome.
Terminator regions are found downstream of genes and often contain regulatory regions affecting gene expression.
Tissue culture refers to a range of techniques used to grow plant cells or explants under sterile conditions on nutrient media.
T-DNA or Transfer-DNA is the section of DNA transferred from Agrobacterium into the plant cell and integrated into the plant genome.
ZFNs (Zinc finger nucleases) are fusions between a zinc-finger DNA binding domain and a nuclease such as Fok1. Similar to TALENs, they can be used to make precise changes within the plant genome.
Introduction
Genetic modification (GM) is a technology that allows changes/additions to be made to the DNA of a plant or other organism to give it a new and useful characteristic, theoretically using DNA from any biological source, be it plant, animal, bacterial, fungal or viral.
The technology is very widely used in diverse applications such as cheese production and in the production of medicines like insulin.
However, GM technology has received most attention when used as a crop breeding tool. Here, we specifically consider the use of GM technology in crop plant breeding and look at similarities and differences between GM technology and other plant breeding techniques.
Plant breeders rely on identifying new and novel genetic variation in order to continually develop improved crop varieties.
Variation in crop plant DNA sequences is required that give rise to new and useful characteristics.
Over the history of plant breeding a number of approaches have been used to provide and/or harness the variation needed to develop improved varieties.
This could involve simply selecting the best looking plants for production of the next generation within existing variable populations.
Cross breeding or hybridisation between related plants followed by selection for the required combination of traits is a widely used technique, although some hybridisations are only possible under laboratory conditions and would not occur naturally.
A variety of techniques have been used to deliberately cause changes in the crop plant DNA increasing the available pool of variation, or mutations, so that useful changes can be identified and included in breeding programmes.
These techniques include generating mutations through tissue culture and using either chemicals or radiation to change or mutate crop plant DNA.
More recently, techniques relying on molecular approaches, such as the use of molecular markers, have considerably speeded up the selection of the required combination of characteristics in crop plants.
Genetic modification is also a technique that uses molecular approaches in addition to providing an alternative way to increase the pool of variation available to plant breeders.
Comparing GM technology to conventional breeding technologies, a number of similarities and differences can be identified.
Firstly, during conventional crossing programmes to introduce a required characteristic, large amounts of DNA will be transferred between closely related plants.
For example, if the requirement is to transfer disease resistance from a wild relative to a crop plant, even after extensive back-crossing, the result may be that some undesirable characteristics are transferred along with the desired disease resistance genes, by genes ‘hitch-hiking’ during the backcrossing process.
With genetic modification however, the exact disease resistance gene could be introduced to the crop plant without large amounts of additional unwanted DNA.
Another key difference between GM and conventional technologies is that conventional approaches can only introduce DNA from closely related plants whereas the DNA introduced using genetic modification could come from any source.
In practise however, most of the genes that are useful for improving our crops come from other plants, but which are not necessarily closely related.
Genetic modification and conventional technologies may both require tissue culture steps, for example embryo rescue may be required in conventional breeding strategies and a tissue culture step is normally required for genetic modification.
In addition, both GM and conventional mutation breeding can cause changes to the plant DNA that cannot be precisely predicted. This is considered below.
Various aspects of GM technology have been highlighted over the years in relation to safety concerns. This has included concerns over the presence of specific ‘undesirable’ DNA sequences in GM plants, the presence of antibiotic resistance genes, the exact type of genetic modification, for example silencing of a native gene, as well as general concerns about the unpredictability of the GM process.
Here, GM technology is examined in detail, broken down into its key components and compared to other plant breeding technologies to address the various concerns related to the technology itself. Like any other technology, genetic modification could be used in a range of different ways producing a wide variety of products with very different characteristics.
Therefore, it is important to focus on the final product when considering possible risks and benefits. During the process of evaluating risk and benefit it is vital to consider that everything we do has some associated risk.
In many cases the risk will be negligible and the benefits will be large. In this paper the focus is on GM technology itself, asking whether there are aspects of the technology that have inherent associated risks compared to conventional technologies or whether the technology is essentially benign.
The genetic modification process
The genetic modification of crop plants requires three basic compo- nents. Firstly, it requires a target tissue into which DNA can be introduced.
This target tissue needs to contain individual cells which have the capaci- ty, given the correct culture condi- tions, to regenerate into a whole new plant.
Secondly, a method of introduc- ing the DNA to the target tissue is required, and thirdly a method allowing genetically modified cells to be distinguished from non-genetically modified cells. These three components are considered individually below followed by consideration of common features of the introduced DNA.
1. Tissue culture methods
Plant tissue culture has a very long his- tory with the first experiments successfully maintaining growth and cell divi- sion of plant cell cultures being reported by White (1934) (1).
Since this time, plant tissue culture has been used in many applications from the commercial micropropagation of a range of species through to the production of valuable products such as pharmaceuticals in plant cell cultures.
In some cases, plant tissue culture is used specifically to induce variation. When plants are regenerated from plant tissue culture some individuals may show clear differences.
This is referred to as somaclonal variation and this source of variation was used in plant breeding strategies during the 1980s and 1990s (2).
The level of somaclonal variation observed is often increased as the time in tissue culture is increased. It is also more common when the cultures have gone through a callus phase.
This is a period of disorganised cell proliferation prior to the formation of organised embryogenic structures capable of regeneration into whole plants.
Figure 1A shows examples of both disorganised callus growth and plant regeneration on a single culture plate.
A variety of different plant target tis- sues is used as the starting point for genetic modification. These include both mature and immature embryos, callus cultures and cotyledonary explants.
A common target tissue used for many cereal species is the immature embryo. Figure 1 (A) shows the generation of callus from immature barley embryos and regeneration of plants from this callus.
Thousands of barley plants have been regenerated from immature embryo derived cultures and most appear normal and are fully fertile (Figure 1B) (3).
The very long and safe history of the use of a variety of plant tissue culture methods gives us confidence that the use of such techniques as part of a genetic modification process does not give rise to any additional risks.
Concerns about genetic variation that may occur as a result of the tissue culture and regeneration process can be addressed by considering the widespread and deliberate genetic variation created in conventional plant breeding programmes.
As well as promoting such genetic change using tissue culture techniques, widespread genetic change has been induced by mutation using chemicals or radiation.
This procedure, referred to as mutation breeding, can be found in the pedigree of many crop plants grown today and has a long history of safe use.
Mutation breeding can lead to unwanted change as well as useful variation but such unwanted changes are simply not selected for further use in breeding programmes.
Any genetic changes caused during the tissue culture steps of the genetic modification process are likely to be far less than those caused during mutation breeding programmes, and in a similar way, plants with any unwanted changes would simply be discarded.
Past experience therefore suggests that there is no cause for concern over the tissue culture component of the genetic modification process.
2. Introducing the DNA
The second component of the genetic modification process is the actual introduction of DNA to the target tissue.
Over the years many different methods have been employed to introduce DNA to plant cells but two methods have predominated, the ‘gene gun’ and Agrobacterium.
The gene gun works by coating the ‘donor’ DNA onto microscopic gold particles and firing them into the plant target tissue.
The first gene guns relied on blank gun cartridges to provide the propulsive force to deliver the DNA coated gold particles.
More recent devices rely on the build-up of helium gas behind a disc that ruptures when a particular pressure is reached. One of the most popular gene guns that relies on helium pressure is shown in Figure 2A.
Although the gene gun has been used very successfully to produce some of the GM crops grown today, the alternative method using the soil bacterium, Agrobacterium, is now far more popular.
Agrobacterium is a naturally occurring bacterial pathogen, and is responsible for causing crown gall disease in a range of plant species.
During the normal infection process, the bacterium is able to transfer a section of its own DNA into the plant where it is stably incorporated into the plant DNA.
This ‘transferred’ or T-DNA contains genes that cause the disease symptoms and the formation of the gall typical of crown gall disease.
In effect, the plant responds to the infection by providing the bacteria with everything they need for survival. In order to harness the ability of Agrobacterium to transfer DNA to plant cells, scientists have removed the genes involved in causing the disease symptoms but left the bacterium with the ability to transfer DNA.
Therefore, if the gene to be introduced to the plant target tissue is placed within the T-DNA then it will be transferred to the plant cells if the target tissue and the Agrobacterium cells are cultured together.
The process of inoculating barley immature embryos with Agrobacterium is shown in Figure 2B. After inoculation, the immature embryos and bacteria are cultured together for 3 days to allow DNA transfer to occur.
The T-DNA is bor- dered by specific repeat sequences referred to as the left and right border regions and in most cases; everything between these border regions is transferred to the plant. These T-DNA border regions can be seen in Figure 3.
There are a number of reasons for the popularity of Agrobacterium as a method of DNA delivery to plant cells in preference to using the gene gun.
These include the fact that Agrobacterium is far more likely to integrate a single copy of a ‘donor’ gene into the plant genome, and DNA delivery is often more efficient.
In addition, there are less re-arrangements of the introduced DNA when it is integrated into the plant genome using Agrobacterium, and expression of the introduced gene is more reliable through generations (4).
Considering Agrobacterium as the preferred method of DNA delivery, it is possible to ask whether there are specific risks associated with the DNA transfer process.
Firstly, it is worth remembering that Agrobacterium-mediated DNA transfer occurs frequently in nature and Agrobacterium has been referred to as nature’s own genetic engineer.
However, transfer of DNA by Agrobacterium is not always precise, regions of DNA from outside of the T- DNA can be transferred to plants, and there have even been reports of Agrobacterium chromosomal DNA being transferred to plants (5).
As this imprecise transfer has been observed during the genetic modification process, it is almost certainly happening during natural Agrobacterium infection.
If additional, unwanted sequences are transferred along with the gene of interest, then these can easily be detected during the molecular analysis of the GM plants and such plants would not be selected for further use.
Another aspect of the DNA delivery process that needs to be considered is the exact site within the plant DNA where the new gene is inserted.
In most GM plants produced to date, it has not been possible to control the insertion site of the DNA and it can be integrated at random into different possible locations in the plant genome; however see the section on new technologies below.
The random nature of the DNA insertion leads to some uncertainty and variability between individual GM plants containing the same introduced gene.
There have been concerns that the GM process might lead to unanticipated consequences, for example the production of toxins or allergens within the GM plants.
There has been a vast amount of research in this area using a range of techniques to examine GM plants for such unexpected consequences of the genetic modification process.
Despite 20 years of research, no evidence for unanticipated changes, within GM plants, that could have safety implications have ever been found (6).
Therefore, considering the biology of Agrobacterium, the detailed molecular analysis that GM plants are subjected to, and the vast scientific literature on the analysis of GM plants, the conclusion of extensive research to date is that the DNA delivery part of the genetic modification process does not pose specific risks.
3. Selecting genetically modified plants
The third and final part of the genetic modification process is the selection of genetically modified cells within the target tissue followed by the selection of regenerated GM plants.
This requires the modified cells and tissues to have a selective advantage over those that are not modified. In order to achieve this, a gene conferring resistance to an antibiotic or to a herbicide is introduced along with the gene of interest.
The relevant antibiotic or herbicide is then included in the nutrient medium on which the target tissue is grown. Only those cells that contain the introduced antibiotic or herbicide resistance gene will be able to grow and regenerate plants on the defined medium.
Antibiotic resistance genes are most commonly used for the selection of GM plants and this has led to concerns about the spread of such genes in the environment.
Two antibiotic resistance genes most commonly used in GM technology are the nptII gene conferring resistance to kanamycin and the hpt gene conferring resistance to hygromycin B.
Both of these genes belong to a group of antibiotics with no or limited therapeutic relevance (7). In addition, both genes are already widespread in the environment. The European Food Safety Authority has concluded that the use of these genes as selectable markers in GM plants does not pose a risk to either human or animal health or to the environment (8).
Studies looking at possible aller- genicity of the protein encoded by the hpt gene conclude that it has a very low allergenic potential (9).
Although the assessment is that these genes do not pose a risk, if they were not present in the final GM plant then any possible concern would be removed.
It is now possible to produce GM plants that do not contain selectable marker genes and this is further considered under technology advances below.
Herbicide resistance genes can also be used as selectable markers, for example the pat or bar genes. These genes give resistance to the glufosinate group of herbicides.
Regulatory authorities in at least 11 countries have approved the release of GM plants containing these genes. Risk assessments have concluded that the presence of these genes does not lead to any meaningful risk to the environment (10).
Herbicide resistant crops can be developed using non-GM as well as GM techniques and in both cases, the use and management of these crops needs careful consideration so as not to complicate the control of weeds or volunteers.
It is possible for herbicide resistant weeds to develop where a specific herbicide is widely used with either conventional or GM crops.
This is however outside the scope of this paper as it is an issue with the use of specific genes, varieties and weed control strategies rather than with GM technology itself.
4. Features of the introduced DNA
When considering the actual DNA transferred to the plant during the genetic modification process it is worth making a few general comments and observations about DNA.
DNA itself is not harmful and since it is present in all living and dead tissues of animals and plants, it is consumed by humans and animals as a normal component of diet in most types of food.
The plant genome, that is the complete set of genetic information for that particular plant, is not fixed and stable.
There are a number of mechanisms that can alter the stability of genomes in non-GM plants (11). Genomes contain very large numbers of mobile (transposable) elements.
Movement of such elements within the crop plant genome cause genetic change and it is likely that this is the source of some of the useful genetic variation selected by plant breeders.
Mutations are very common in crop plants and a comparison of different varieties of the same crop plant will reveal large numbers of differences in the DNA sequence.
There is evidence, in most genomes, of the transfer of DNA from another species at some point in the past. This is referred to as horizontal gene transfer and it occurs without reproduction or human intervention (7).
Often the transfer of genes by horizontal gene transfer involves viruses or mobile elements. The observed frequency of the transfer of genes from plants to other organ- isms is extremely low.
In addition to the natural sources of variability mentioned above, the environment that a plant is grown in will have a large influence on the activity of a range of its genes.
Therefore, it is important to consider this natural variability and instability in non-GM plants when assessing whether DNA introduced during the GM process leads to any additional instability and associated risk.
There are a number of features of the actual introduced DNA that are common to different GM crop plants. As described above, when Agrobacterium is used to introduce the DNA, the expectation is that everything between the right and left borders of the T-DNA is inserted into the crop plant.
Figure 3 shows a typical T- DNA from a transformation vector and the entire transformation vector is shown in Figure 4.
The gene of interest and the selectable marker gene can be seen between the T-DNA borders of T-DNA 1, together with the necessary control regions; promoter and terminator for each gene.
The complete vector is a circular or plasmid DNA that can be easily manipulated in safe laboratory strains of E. coli and can also be transferred to Agrobacterium.
Vectors for genetic modification have to be built in the laboratory using a range of molecular tools, manipulated and multiplied up in E. coli.
Certain regions of the vector are required to allow it to be maintained in E. coli and in Agrobacterium. These regions are found outside of the T-DNA as they do not need to be transferred to the plant.
Here, the different component parts of the vector are considered together with the possible risks associated with each part.
Firstly, the regions of the transformation vector outside of the T-DNA are considered because it is possible for these to be transferred to the plant during the genetic modification process.
These regions are often referred to as ‘backbone sequences’. They include a bacterial selectable marker to allow selection of bacterial cells containing the correct vector and bacterial origins of replication that allow the plasmid to be replicated in the bacterial cells.
The DNA regions commonly found in the backbone of transformation vectors are found in bacterial populations in the environment.
They do not therefore present particular additional hazards when present in GM plants. However, GM plants being considered for release to the environment or for food or feed use are thoroughly analysed to ensure that no vector backbone regions are present.
This analysis is now very straightforward and routine due to advances in molecular analysis tech- niques meaning that the presence of unwanted additional DNA sequences in GM plants should no longer be an issue of concern.
In most GM crop plants produced to date, a gene or genes of interest are expressed under the control of specific promoters (Figure 3) and produce a new protein in the GM plant.
The pro- tein produced by each gene of interest, and the resulting novel characteristics of the plant, must be subject to appropriate risk assessment on a case by case basis.
Any risks associated with a particular gene will be specific to that genetic modification event only and are not considered here.
A second, less common, type of genetic modification allows the activity of a gene already present in the crop plant to be reduced or switched off. In these genetic modification events the transformation vector is designed to lead to the production of a double stranded RNA (dsRNA).
The presence of dsRNA in the plant leads to a process called RNA interference or RNA-induced gene silencing (RNAi) which is a method whereby RNA produced by a target gene is degraded.
This effectively silences or switches off the target gene. The process is now known to be vital in controlling gene expression and is also important in protecting plants from virus attack.
RNAi approaches have been used to produce GM crops resistant to disease and with enhanced nutrient content.
The first commercialised genetically modified plants, Flavr Savr tomatoes, that had a longer shelf life than non-GM tomatoes, made use of RNA interference to reduce activity of a target gene.
Similar to DNA, RNA and dsRNA are normal components of our food and RNA interference is a natural mechanism occurring in both plants and animals.
As dsRNAs are important regulatory molecules, it has been suggested that additional risk assessment procedures might be needed for this type of GM technology (12).
However, the case for this is again based on the possibility of adverse, unanticipated effects of the genetic modification.
The vast amount of research looking for, and failing to find, such unanticipated effects has already been mentioned above.
Each GM event using RNAi technology to produce a specific dsRNA must be assessed for any risk specific to the particular dsRNA in a similar way to the assessment of GM plants expressing a particular gene of interest.
There is no evidence to suggest that RNAi-based GM events should be treated any differently to other GM events.
In both types of genetic modification mentioned above, it is necessary to drive the gene, or gene region giving rise to the dsRNA, with an appropriate promoter.
Promoters can control the level of expression of a gene as well as sometimes controlling the exact parts of the plant where the gene is active or the exact time during plant development when the gene is switched on.
Promoters that are generally active in all parts of the plant at all times are referred to as constitutive promoters.
Other promoters may be tissue specific, developmentally specific or inducible in some way (Figure 3). There are certain promoters that have been extensively used in the production of GM plants including the CaMV 35s promoter.
This promoter is derived from cauliflower mosaic virus and is a strong constitutive promoter. The CaMV virus infects mostly plants of the Brassicaceae family such as caulifower.
The fact that the promoter originates from a virus has led to some concerns. However, as previously mentioned, viral sequences can be integrated into plant genomes by the process of horizontal gene transfer so the presence of such sequences in plants does not only occur through GM approaches.
Genome sequencing projects are now revealing more examples of horizontal gene transfer indicating that it is a highly significant process in genome evolution (13).
A recent paper highlighted the fact that some versions of the CaMV 35s promoter contain fragments of a viral gene (gene VI) (14).
The issue of unintended effects, including the possible production of unintended proteins in the GM plant was again raised.
The authors concluded that there was no risk of toxic or allergenic proteins being produced due to the presence of gene VI.
The safety of the CaMV 35s promoter has been considered in the literature over many years. Hull et al. (2000) (15) assessed the possible risks and concluded that using the 35s promoter in GM plants posed no greater risk than that encountered using conventional breeding techniques.
The CaMV 35s promoter is used in many of the GM crops currently being grown and has therefore been subject to stringent risk assessment and has a long history of safe use.
These facts, together with our increasing knowledge of the plasticity of plant genomes leads to the conclusion that use of this promoter does not raise any cause for concern.
Technology advances
There have recently been a number of important advances in genetic modification technology that further diminish remaining concerns.
Many of the concerns mentioned above have the same foundation; worry that the GM process may lead to some unintended and unanticipated outcomes.
Much of this uncertainty is linked to the random nature of the insertion of the introduced gene. Even though the exact insertion site of the introduced DNA can be determined and analysed, this uncertainly remains.
In practice, for any individual gene introduced to a crop plant, many independent genetically modified plants will be made and then those with the required characteristics will be selected. In this way, any negative effects linked to the exact insertion site would be discarded.
However, recent advances now mean that genes can be inserted into predetermined, precise locations within the plant genome. As well as allowing insertion into precise locations, these new technologies allow changes such as specific mutations, to be made at particular locations.
The term used to cover these new technologies is genome engineering or genome editing and they rely on the use of sequence-specific nucleases.
The two most common classes of nucleases are zinc finger nucleases (ZFNs) and transcription activator-like effector nucleases (TALENs).
In both cases the nucleases create double strand breaks in the plant DNA at a precise location leading to either, an insertion, deletion or precise change to the DNA sequence (16). Although these tech- nologies are not yet in routine use, they are being very quickly adopted.
Additional technology advances that increase the precision of the genetic modification process include the use of promoters that give expression of the introduced gene exactly where and when it is required.
The range of specific promoters available for use in crop plants continues to grow thus allowing improved transformation vectors to be designed and produced.
Promoter choice during the construction of the transformation vector is illustrated in Figure 3. Advances to transformation vectors also allow GM plants to be produced that do not contain a selectable marker gene.
Antibiotic resistance genes are required during the genetic modification process to allow plants containing the new genes to be selected.
However, it is now relatively easy to use a selectable maker during the GM process but to ensure that it is not present in the final GM plant. This is most simply achieved by introducing the gene of interest on one T-DNA and the selectable marker gene on a second T-DNA.
Figure 3 illustrates this approach by showing the selectable marker gene on T-DNA 2 and the gene of interest on T-DNA 3.
In some cases these genes will be integrated into the plant DNA at different locations. This means that they can be separated in the next generation by normal genetic segregation, and plants with only the gene of interest selected for further use.
Improved design of transforma- tion vectors also allows the number of GM plants containing backbone vector sequences to be reduced, simplifying the analysis of the resulting plants.
Taken together, these technology advances, in particular the ability to make precise changes at a predetermined location within the plant DNA, remove many of the concerns highlighted in the sections above.
Conclusions
Without new sources of variation in crop plant DNA, it would not be possible to improve crops to tackle the many challenges facing us in providing sufficient food in a sustainable way.
Genetic modification is one way of providing such variation. By breaking down genetic modification technology into its component parts, many similar- ities with other technologies used in crop improvement and with natural processes can be found.
Tissue culture methods have been used in a range of conventional approaches to produce commercial crops.
Tissue culture has even been used specifically to induce useful variation. Other methods widely used in conventional breeding programmes to induce mutation within DNA include the use of chemicals or radiation in mutation breeding strategies.
These methods, leading to multiple random changes to crop plant DNA, have not lead to crops being produced that are unsafe.
The recent increase in the availability of plant genome sequences has further highlighted the plasticity of genomes with mutations being common and large numbers of mobile elements being present.
The GM process has often been criticised for allowing species bar- riers to be overcome and genes to be transferred between species that could not normally be crossed to produce progeny.
However, the natural process of horizontal gene transfer is more widespread than previously thought and has allowed gene transfer between distant species.
Agrobacterium used in the GM process is often referred to as nature’s own genetic engineer. The components of genetic modification technology are very similar to either natural processes or to conventional breeding technologies.
This, together with extensive evidence that the GM process does not lead to unanticipated outcomes with associated risk, leads to the conclusion that GM technology is not a risky method but is instead a valuable tool increasing the pool of variation available to breeders.
Acknowledgements
Mark Smedley is thanked for figure 4. The work was supported by BB/J004588/1 from BBSRC and the John Innes Foundation.
References
1. White P R, (1934) Potentially unlimited growth of excised tomato root tips in a liquid medium. Plant Physiol. 9: 585-600.
2. Karp A, (1995) Somaclonal variation as a tool for crop improvement. Euphytica 85: 295- 302.
3. Bartlett J G, Alves S C, Smedley M, Snape J W, Harwood W A (2008) High-throughput Agrobacterium-mediated barley transformation. Plant Methods, 4: 22.
4. Travella, S, Ross S M, Harden J, Everett C, Snape J W, Harwood W A (2005) A comparison of transgenic barley lines produced by particle bombardment and Agrobacterium-mediated techniques. Plant Cell Reports 23: 780-789.
5. Ülker B, Li Y, G Rosso M G, Logemann E, Somssich I E, Weisshaar B, (2008) T- DNA–mediated transfer of Agrobacterium tume- faciens chromosomal DNA into plants. NatBiotechnol. 26(9):1015-1017.
6. Herman R A, Price W D, (2013) Unintended Compositional Changes in Genetically Modified (GM) Crops: 20 Years of Research. J. Agric. Food Chem., DOI: 10.1021/jf400135r.
7. Keese P (2008) Risks from GMOs due to horizontal gene transfer. Environ. Biosafety Res. 7: 123-149.
8. EFSA (2009). Scientific opinion of the GMO and BIOHAZ panels on the ‘Use of antibiotic resistance genes as markers in genetically mod- ified plants’. European Food Safety Authority doi:10.2903/j.efsa.2009.1108.
9.LuY,XuW,KangA,LuoY,GuoF,YangR, Zhang J, Huang K (2007) Prokaryotic expres- sion and allergenicity assessment of hygromycin B phosphotransferase protein derived from genetically modified plants. Journal of Food Science 72: (7).
10. Center for Environmental Risk Assessment (2011) A review of the environmental safety of the PAT protein. Environ. Biosafety Res. 10: 73- 101.
11. Weber N, Halpin C, Hannah L C, Jez J M, Kough J, Parrott W, (2012) Crop genome plas- ticity and its relevance to food and feed safety of genetically engineered breeding stacks. Plant Physiology 160: 1842-1853.
12. Heinemann J A, Agapito-Tenfen S Z, Carman J A (2013) A comparative evaluation of the regulation of GM crops or products con- taining dsRNA and suggested improvements to risk assessments. Environment International 55: 43-55.
13. Bock R, (2009) The give-and-take of DNA: horizontal gene transfer in plants. Trends in Plant Science 15: 1.
14. Podevin N, Jardin P (2012) Possible conse- quences of the overlap between the CaMV 35s promoter regions in plant transformation vec- tors used and the viral gene VI in transgenic plants. GM Crops and Food: Biotechnology in Agriculture and the Food Chain. 3: 4.
15. Hull R, Covey S N, Dale P (2000) Genetically modified plants and the 35s pro- moter: assessing the risks and enhancing the debate. Microbial Ecology in Health and Disease 12: 1-5.
16. Curtin S J, Voytas D F, Stupar R M (2012) Genome engineering of crops with designer nucleases. The Plant Genome 5:2.
Download pdfFigures
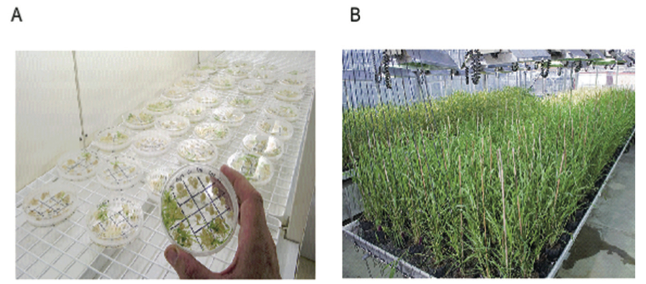
Figure 1. A) Regeneration of genetically modified barley plants from cultures derived from immature embryos. Some of the immature embryos are developing green shoots on selective medium showing that they are genetically modified, while others have only produced small amounts of callus and are not genetically modified. B) Genetically modified barley in the glasshouse showing uniform growth and full fertility.
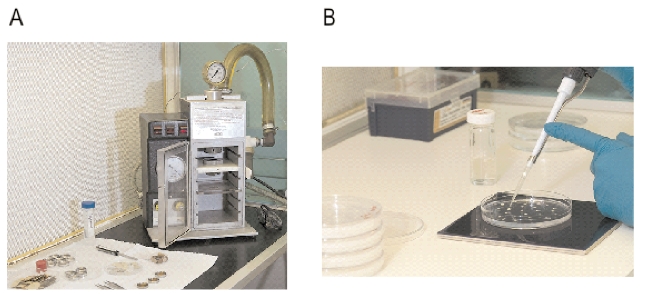
Figure 2. Introduction of the DNA to target tissues. A) The gene gun. This device delivers microscopic gold particles coated in DNA into plant target tissue. The propulsive force is provided by release of heli- um gas following the rupture of a disc at the base of the gun barrel. B) Inoculation of immature embryos with Agrobacterium. Following inoculation, the target tissue is co-cultivated with the Agrobacterium for 3 days during which time DNA transfer takes place.
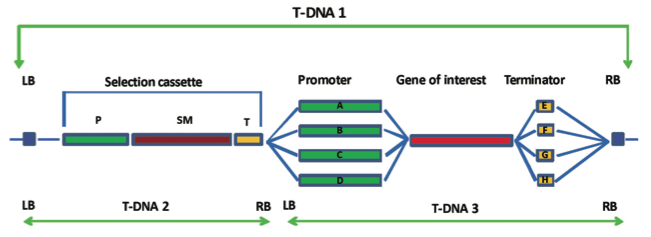
Figure 3. Diagrammatic representation of construction of the T-DNA region of a transformation vector. In T-DNA 1, all components are within the T-DNA border regions (LB and RB). The selection cassette contains a selectable marker gene (SM), often an antibiotic resistance gene, under the control of a promoter region (P) and a terminator region (T). The gene of interest may be controlled by a constitutive promoter leading to expression of the gene in all tissues (A), a tissue specific promoter giving expression in a specific tissue only (B), an inducible promoter that is activated for example by stress (C) or a development stage specific promoter only active at a particular devel- opmental stage of the plant (D). Similarly, different terminator regions can be used (E-H). It is possible for the selection cassette to be present on a separate T-DNA (T-DNA 2) to the gene of interest with its associated control regions (T-DNA 3). In this case, offspring plants can be selected that contain only the gene of interest with no selectable marker.
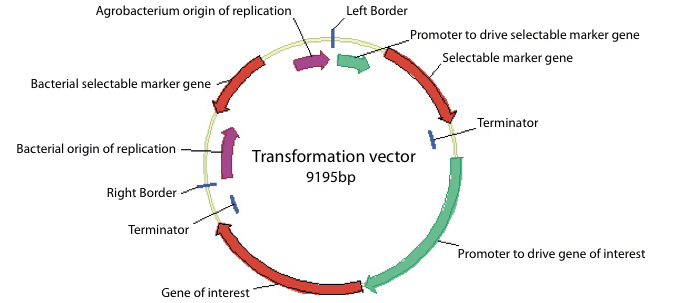
Figure 4. Map of a typical plasmid used for genetic modification showing the common components. The T-DNA region detailed in figure 3 is shown between the right and left T-DNA borders. The sequences present on the backbone of the plasmid are also shown including the bacterial origins of replication and the bacterial selectable marker gene.