An holistic approach to understanding the biological impacts of climate change: Antarctica as a planetary warning system
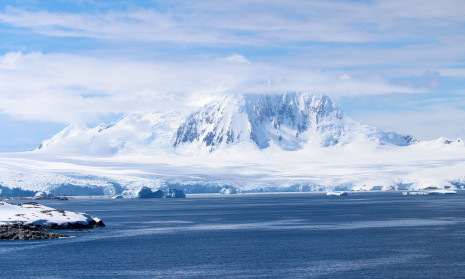
British Antarctic Survey, Natural Environment Research Council, High Cross, Madingly Road, Cambridge CB3 0ET, UK
Index introduction
A definitive and comprehensive paper assessing the effects of climate change on the terrestrial and marine flora and fauna in Antarctica.
The review explores the lessons that can be drawn from this regional climate and the need to understand the interactions between different anthropogenic causes of environmental change.
Antarctica provides records of change preserved in ice and sediments which provide evidence of natural patterns of subdecadal and longer-term patterns.
It is an important natural laboratory to study and identify contemporary human influences on the ecological consequences of change.
Summary
In addition to warming, the northern Antarctic Peninsula, associated archipelagos, and some sub-Antarctic islands are also experiencing a rapid increase in de-glaciated terrain and changes in precipitation and irradiation patterns.
The newly available land provides fresh but immature habitat for colonisation by land plants: combined with the influence of climatic warming and changes in patterns of water availability, some have undergone a rapid increase in abundance and changes in the utilisation of life history strategies.
The changes in climate have more subtle effects on terrestrial animals and, while responses to or consequences of individual elements of climate change can be identified at species or individual level, as yet few attempts have been made to synthesise these across either communities or multiple environmental variables.
In addition to consequences attributable to environmental change, Antarctic ecosystems now also face the direct impacts of human presence, in the form of increasing incidence of introduction and establishment of non-indigenous ("alien") species.
Direct (introduction) and indirect (via environmental change) consequences of human action will act synergistically to further impact native ecosystems, through a combination of lowering the barriers to successful colonisation and establishment and increasing the frequency and diversity of biota introduced to the region.
To date, most marine and oceanic habitats have shown only equivocal evidence of warming, but there has been a marked decrease in sea ice extent in the region west of the Antarctic Peninsula, most recently linked with evidence of rapid warming at near-surface (< 100 m) depths.
Antarctic Peninsula regional warming, decreasing sea ice extent, and some biological parameters, are linked through global teleconnection to ENSO events.
Contemporary marine environmental changes, while small in absolute terms, are likely to be sufficient to influence the population dynamics of many marine organisms, and also approach the tight ecophysiological stress tolerance limits of some, as strong stenothermy is often a feature of their biology.
Southern Ocean marine ecosystems have, however, already been heavily and possibly irreparably impacted by the direct effects of human economic activity.
Key words
Antarctica, colonisation, cross-disciplinary, ecosystem, environmental change, human impact, non-indigenous species, synthetic approach, trophic interactions.
Glossary
ACC: Antarctic Circumpolar Current — an eastward moving oceanic current surrounding the Antarctic Continent in the Southern Ocean
ENSO: El Nino Southern Oscillation
Gene chip (or “microarray”): A glass microscope slide that is coated with 10005 of minute spots, each of which contains DNA representing a gene from a particular organism(s). Using this technology, we can screen the activity of large numbers of genes in relation to changes in environmental conditions.
NIS: non indigenous species - an "alien" organism that does not form part of the native biota of a defined region.
PAR: photosynthetically active radiation, 3 term used to describe the wavelength range (ca 400 - 700 nm) of visible light that can be absorbed by the various pigments involved in photosynthesis, providing the energy to permit the fixation of carbon from atmospheric carbon dioxide into organic molecules (sugars).
Poikilothermic: an organism that does not generate sufficient heat internally to allow maintenance of a body temperature different from that of its environment.
Stenothermy: term used to describe an organism that can survive only within a narrow temperature range
UV: ultra-violet radiation
Calcite compensation depth: the ocean depth, usually several km, below which calcite does not accumulate because it is a level on the sea floor at which the rate of carbonate supply is equal to the rate of carbonate dissolution. it is analogous to the snow line on land.
Introduction
The Antarctic is remote from most human experience and activity, even today, and it is only a little over a century since a person first set foot on the continent.
However, it is not remote from the consequences of human actions, a fact spectacularly illustrated not least by the exceptional rates of climate change seen in parts of the region over the last 50 years or so, but also by its exposure each austral spring since the early 1980s to the ”ozone hole", a separate consequence of anthropogenic pollution of the upper atmosphere.
Between these two major environmental perturbations, four changing environmental variables can be predicted to have a direct influence on Antarctic biota: temperature, water {to the terrestrial environment, through precipitation and melt), solar radiation (PAH and UV) and atmospheric CO2 concentrations.
The first three have been the focus of research in the Antarctic. In contrast, although increasing levels of CO2 are recorded across Antarctica as across the planet, its biological consequences have not been directly addressed in the region. Now, despite its remoteness, Antarctica and its biology is, through its exposure to rapid environmental change, becoming a "canary in the coal mine" (Convey, Scott & Fraser, 2003) providing fundamental biological lessons applicable to the rest of the planet.
The field of climate change is obviously far more complex than the often used (and misused) term “global warming” would imply.
However, it is now generally accepted that one important element of climate change — the recent warming trends evident in global and many regional data records — is both real and linked intimately with the rapid increase in the release of “greenhouse" gases (particularly CO2) into the atmosphere as a result of human activity since the industrial Revolution (King 2005).
Most attempts to model this warming process, notwithstanding justifiable caveat and recognition that contemporary models fall short of real world accuracy (eg. King et al, 2003), closely agree in predicting enhanced rates of climate change, particularly temperature increase, at higher latitudes.
The Antarctic terrestrial and limnetic environments, with their extremely low indigenous biodiversity linked with severe environmental stresses, geographical isolation and the youth of most habitats, are currently faced with rates of climate change amongst the most rapid on the planet (Quayle et al. 2002, Vaughan et al. 2003, Bergstrom. . 2006), with the latest analyses showing increases of 0.56°C decade-1 over the last 50 years (Turner . 2005a).
Polar ecosystems are expected to show particular sensitivity and rapid biological responses (Freckman & Virginia 1997, Quayle et al. 2002, 2003), and were singled out by the Inter— governmental Panel on Climate Change (lPCC) Third Assessment (lPCC 2001) as areas of special concern.
The severe conditions of the Antarctic terrestrial environment have ensured that to date it has not been a focus of economic activity, although throughout the Twentieth Century attempts have been made to establish agricultural outposts on some sub-Antarctic and more northern cold temperate oceanic islands (eg. Headland 1984).
While not being economically viable, these have served both wide-scale political purposes and enhanced local recreational opportunities or quality of life for the transient human populations of these islands. Their main tangible consequence for Antarctic biology has been the introduction and establishment of a wide range of non—indigenous vertebrate herbivores and predators, invertebrates and plants, some of which have had, and continue to have, considerable deleterious impacts on native biota and ecosystems (Frenot et al. 2005).
In contrast, the Southern Ocean marine ecosystem has been a focus of economic activity since the discovery of large fur seal (Arclocephalus gazella) populations in the late Eighteenth Century.
The history of overexploitation and destruction of stocks of seal and successive whale species is hardly glorious (Fig. 1; see, e.g., Headland (1984) and Basberg (2004) for descriptions of the history of these industries on South Georgia) but today, through the auspices of the Convention for the Conservation of Antarctic Marine Living Resources (CCAMLR, an international convention agreed by the signatories of the Antarctic Treaty), at least the ongoing legal Southern Ocean fisheries for krill, squid and various fish species are probably the most effectively monitored and regulated in the world.
In this critical review, our aim is to draw together diverse strands of contemporary Antarctic biological research, placed in a context of ecosystems subject to the most rapid rates of regional climate change currently occurring on the planet.
We highlight biological messages that can be drawn out from the Antarctic and used to improve understanding of actual and likely consequences of environmental change worldwide, both for natural and managed ecosystems.
Our approach, necessarily selective and synthetic, builds on a palaeobiological foundation and considers responses from the level of the genome upwards through levels of complexity including organism physiology, life history strategy and populations, and community and ecosystem processes.
Antarctic climate history
Climate change has occurred throughout the Earth’s history. The Earth was in a state of extreme global warmth from the Cretaceous (144 to 65 million years (ma) ago) to the early Eocene (ca 55 ma), during which time Antarctica was part of the supercontinent Gondwana, with a terrestrial fauna and flora typical of south temperate rainforest regions (Poole & Cantrill 2001).
With the opening of the Drake Passage (30—35 ma) (Livermore et al. 2005) and the separation of the Tasman Rise from Antarctica (33.5 ma) deep water circulation became possible around the Antarctic.
The resulting Antarctic Circumpolar Current (ACC) isolated a cool body of water (the Southern Ocean), established a polar front and accelerated a major cooling of the Antarctic continent. By the middle to late Eocene (42 ma) there were a series of several small glaciations and one major transient glaciation of the Antarctic.
Studies of the oxygen isotope composition of marine calcite in sediment cores suggest that this “greenhouse_icehouse" transition was closely coupled to the evolution of atmospheric carbon dioxide, and that negative carbon cycle feedbacks may have initially prevented the permanent establishment of large ice sheets (Tripati et al. 2005).
However, by 34 ma, at the Eocene-Oligocene transition, large ice sheets appeared on the Antarctic continent evidenced by decreasing atmospheric carbon dioxide concentrations and a deepening of the calcite compensation depth in the world's oceans coinciding with changes in seawater oxygen isotope ratios.
After 15 ma, a further cooling is believed to have caused the transition from an ephemeral to a permanent Antarctic ice sheet (Barret 2003).
Since the formation of the Antarctic ice sheet, the continent’s climate has been far from stable. During the most recent geological period, the Quaternary, that spans approximately the last 2 ma, the polar ice sheets developed their characteristic cycle of slow build up to full glacial conditions, followed by rapid deglaciation to interglacial conditions (Williams et al. 1998).
Even within the interglacials there have been periods of lower and higher temperature, for example the optima at ca 11,500-9,000 cal yr BP (Masson et al. 2000) and 3,800-1,400 cal yr BF (Hodgson & Convey 2005) in the Holocene.
The broader glacial— interglacial changes in the configuration of the ice sheets are largely driven by the cyclical changes in the Earth’s orbital path around the sun, controlling the amount of solar radiation reaching the Earth (Milankovitch cycles].
The most influential of these are the 41 kyr (thousand years) obliquity cycle that paced the Quaternary glaciations prior to 400 kyr ago and the 100 kyr eccentricity cycle that has paced the Quaternary glaciations since 400 kyr (Williams et al. 1998).
However, if glacial-interglacial cycles were solely driven by cyclical changes in the Earth’s orbital path around the sun, then one would expect alternating glaciations in the Northern and Southern Hemispheres, as one, and then the other pole became positioned nearer to the sun.
What has ensured near synchronous glacial behaviour between both poles, despite the difference in their respective positions relative to the sun, are the changing levels of global atmospheric CO2 experienced during glacial cycles.
Carbon dioxide in the atmosphere originates from a variety of sources, for example, the natural decay of organic material, the combustion of organic matter, and aerobic respiration. These natural sources are nearly balanced by physical and biological processes that remove carbon dioxide from the atmosphere.
For example, the oceans exert an important control on global CO2 concentrations, and contain over 60 times more carbon than the atmosphere.
The oceans can remove CO2 from the atmosphere through two processes. the biological pump (which fixes carbon through photosynthesis, respires it back into the ocean, and transports about ten per cent to the deep ocean) and the solubility pump (where cold saline water transfers dissolved CO2 to the deep ocean). Changes in the efficiency of these pumps and their link with the Milankovitch cycles and global atmospheric CO2 is believed to be, in part, due to changes in ocean circulation together with changes in high latitude surface ocean productivity and variations in total ocean nutrient distribution.
Important changes in ocean circulation include the rate of North Atlantic Deep Water (NADW) formation. During glacial stages NADW flow into the Antarctic is reduced and this results in a dominance of up—welled Circumpolar Deep Waters (CPDW) in the surface layers with an increased partial pressure of CO2.
This corresponds to the reduced atmospheric CO2 measured during glacial stages in ice cores. Other factors may also be involved such as sea ice extent limiting out-gassing from the ocean (Stephens & Keeling 2000), and colder ocean temperatures increasing the solubility of CO2.
Whilst the magnitude of the leads and lags between CO2 and atmospheric temperature during different phases of the glacial cycles are debated, several ice core records now show that the atmospheric global (CO2and temperature have been more or less coupled for at least the last 800,000 years.
Thus, the coupling between CO2 as a principle greenhouse gas (along with other greenhouse gases such as (CH4 and N20) and global temperature is well established (Houghton 2007, King 2005). Modelling studies also confirm that CO2 variations have an important amplifying effect on the glacial- interglacial cycles (Weaver et al. 1998).
Under natural conditions the level of CO2 in the atmosphere during the Quaternary glacial - interglacial cycles has varied between 180 and 300 ppm.
Through the burning of fossil fuels, largely since the Industrial Revolution, humans have driven the level beyond the bounds of this natural variability, currently to about 380 ppm, with a rise of about 20 ppm over only the last ten years (Fig. 2).
The result is that atmospheric CO2 is now at levels about 30% higher than at any time in the Quaternary, and methane 130 per cent higher, and that the contemporary rates of increase are exceptional – for CO2, 200 times faster than at any time in the last 650,000 years.
The consensus amongst most scientists {Houghton 2001, King 2005) is that these increases in CO2 and other greenhouse gases are causing global temperatures to rise, forcing the Earth toward a new and potentially unsteady state. This increase in temperature has not been uniform across the globe but has been particularly focused on the polar regions.
Contemporary Antarctic climate change
Since the 1950s the western Antarctic Peninsula and associated island archipelagos has been one of the three most rapidly and consistently warming areas of the world (Fig. 3; Smith 1990, Skivarcs et al. 1998, King et al. 2003, Vaughan et al. 2003, Turner et al. 2005a), notwithstanding considerable interrannual variability.
At Faraday/Vernadsky Station (Argentine Islands, ca 65°S) mean annual air temperature has increased by 0.56°C decade-1 (Vaughan et al. 2003, Turner et al. 2005a).
Warming trends are also seen at several sub-Antarctic islands (Bergstrom & Chown 1999) and at some coastal locations of continental Antarctica, although much of the continental area shows no clear trend or even local cooling (Doran et al. 2002).
The rapid increases along the Antarctic Peninsula seem to be linked locally with decreasing winter sea ice extent and, on a larger scale. to El Nino Southern Oscillation (ENSO) events in the southern Pacific Ocean (Cullather et al. 1996, Harangozo 2000).
Recent analyses of long-term datasets of life history parameters of Southern Ocean megafauna have likewise detected a link with environmental variability and ENSO events (Forcada et al. 2005, 2006).
Until recently, these temperature trends have not been extended to include detectable effects in the surrounding seas. However, Meredith & King (2005) have now reported an analysis of oceanographic data indicating that surface (< 100 m depth)waters west of the Antarctic Peninsula have also warmed by as much as 1°C over the last 50 years, along with changes in salinity, although there was no detectable effect at greater depth.
Air temperature trends may vary both throughout the year and with location. Along the western Antarctic Peninsula the strongest trend is due to warming during the winter (11 ± 9°C per century), with much less (but still significant) warming in summer (2.4 ± 1.7oC per century) (King & Harangozo 1998, King et al. 2003, Vaughan et al. 2003). in contrast, at Signy island (South Orkney Islands) most warming has occurred during the summer (Smith 990, Convey et al. 2003).
Such differences between local trends may have considerable biological significance (reviewed by Convey 2003, 2006) at least in terrestrial ecosystems, as they influence the length of the active season, and the timing of release and duration of presence of liquid water and nutrients.
The potential biological significance of the changes seen to date is emphasised by the use of a measure of physiological time (day degrees above 0°C — a measure integrating the length of time and amount by which temperature remains positive).
Again, using data from Faraday/Vernadsky Station, Vaughan (2006) has shown a highly significant 74 per cent increase in this measure over the last 55 years, equating to approximately 77 day degrees.
It has separately been shown that increases of only 100-300 day degrees per season are sufficient to generate major shifts in life history of some polar invertebrates (Strathdee et al. 1993, Arnold & Convey 1998).
If temperature is a highly noticeable changing environmental variable, the availability of liquid water is thought to be an even more fundamental factor influencing the biology of many polar terrestrial biota (Kennedy 1993, Somme 1995, Block 1996).
Although currently impossible to model or describe at an appropriate spatial scale, it is clear that precipitation is linked with other variables (e.g. insolation — including receipt of PAR and UV, cloud cover, wind speed), while temperature obviously influences its form (rain vs snow).
Meteorological records show a general increase in precipitation in coastal areas of the maritime Antarctic (Turner et al. 1997, 2005b), and also that, especially in the northern maritime Antarctic, summer precipitation increasingly occurs as rain (Quayle et al. 2003) and therefore becomes available immediately to terrestrial biota.
Decreases in precipitation are also being reported from some Antarctic locations, particularly in the Sub-Antarctic (Bergstrom & Chown 1999; Convey 2006).
Although an important source of water to terrestrial ecosystems, precipitation in the form of snow, or onto frozen ecosystems, does not become immediately available to biota.
Subsequent snowmelt and the melting of glacial ice are the normal routes by which water enters terrestrial ecosystems.
In this context, recent rapid glacial retreat, along with loss of permanent snow cover, become significant (e.g. Smith 1990, Fox & Cooper 1998, Kiernan & McConnell 2002, Skvarca & De Angelis 2003).
Warming trends and earlier melt also raise the possibility of exhaustion of available ice or snow, paradoxically thereby increasing rather than reducing water stress on terrestrial biota during the summer period (see Convey et al. 2003). More subtly, recent evidence of rapid changes in salinity in some coastal continental Antarctic lakes is interpreted as indicative of a change in the balance between water input to the lakes and evaporation and sublimation (Hodgson et al. 2006).
The final environmental variable for which change processes are of fundamental biological relevance is solar radiation. Direct insolation is clearly inseparable from the environmental variables already discussed, with any changes (e.g. through changing cloud cover) likely to alter primary production, the fundamental basis of most ecosystem processes.
Separately, since the early 19803, depletion of the stratospheric ozone layer (Farman et al. 1985) has altered spectral composition and intensity of incoming radiation, causing increased exposure to damaging shorter wavelength UV radiation (UV-B) for terrestrial and shallow marine biota.
Levels of exposure to UV-B at ground level under the ozone hole are similar to those normal in mid-summer, and its biological significance lies with the fact that these occur much earlier in the season than normal (Fig. 4), at a time when many terrestrial organisms have not resumed normal physiological activity and remain in their winter frozen or desiccated and inactive state.
Ozone depletion is caused by a separate anthropogenic pollution process to that underlying warming, although the two share a common influence relating to the strength of the upper atmospheric polar vortex (Thomson & Solomon 2002).
However, the consequential impacts of increased UV-B radiation on biota are also modulated by other environmental variables, particularly cloud cover and type (Gautier et al. 1994, Sabburg & Wong 2000), and even the presence and properties of thin layers of snow (Cockell et al. 2002).
Life history strategies and population changes
The most frequently cited example of a biological response to environmental change in the Antarctic is that of the continent’s only two native flowering plants (the grass, Deschampsia antarctica, and the pearlwort, Colobanthus quitensis).
Very rapid increases – up to two orders of magnitude – in their populations (numbers and local extent) have been reported in studies in the maritime Antarctic (Fowbert & Smith 1994, Smith 1994, Grebe et al. 1997, Gerighausen et al. 2003).
It is thought that increased temperatures have encouraged growth and vegetative spreading. And increased the likelihood that germinating seedlings will develop sufficiently to permit establishment.
Separately, warming has led to a greater frequency of successful sexual reproduction (Convey 1996a), and is reported to stimulate growth of seeds from soil propagule banks (McGraw & Day 1997).
There have yet to be comparable quantitative and non—manipulative field studies of either the bryophyte flora (the dominant vegetation type in the maritime Antarctic) or of the invertebrate fauna of the habitats provided by the plants.
Qualitative observations support a similar increase in local scale extent and abundance of bryophytes, and also that newly available vegetated habitats are rapidly colonised by an invertebrate fauna typical for the location concerned.
Exceptional flexibility is a general feature of the life history strategies of many Antarctic terrestrial organisms {Convey 1996b, 1997, 2000), and potentially allows accelerated development and shortening of the life cycle in response to improving environmental conditions.
Although there are few experimental demonstrations of this occurring, the sub—Antarctic diving beetle, Langustis angusticoflis, is a species likely to show a rapid response, as the levels of warming being experienced in its natural habitat are already almost sufficient to permit a step change in its life cycle from biennial to annual (Arnold & Convey 1998).
As the top predator in its ecosystem, any such change will have a large though unpredictable impact on local trophic dynamics. An analogous step change in life history characteristics (in this case of an aphid) has been described in a manipulation study of an Arctic tundra habitat (Strathdee et al. 1993).
In the context of understanding responses to changing patterns of water availability, a meter practical problem to be faced both in the Antarctic and elsewhere relates to the difference in physical scale between that of meteorological and micrometeorological monitoring equipment and that at which an individual organism operates, particularly in soil habitats.
Thus, in the only study of the water relations of maritime Antarctic micro-arthropods (mites and springtails), Block & Convey (2001) and Convey et al. (2003) used an alternative approach, treating ecophysiological data as a proxy measure of environmental conditions, and identifying patterns in animal water content that suggested consistency with local climate trends.
With the limited availability of quantitative field data, extended (months to years) field manipulation studies involving passive cloches or screens (often described as greenhouse methodologies) have been used to imitate the predictions of climate change and attempt to identify likely responses (Fig. 5).
Such studies in the Antarctic uniformly result in very rapid responses (in terms of increased population densities) across the bryophyte and microbial flora and the invertebrate fauna (Smith 1993, 2001, Kennedy 1994, Wynn-Williams 1996a, Convey & Wynn-Williams 2002), and also illustrate the importance of dormant propagule banks in the rapid establishment of communities (to date consisting only of local species) in manipulations on previously visibly bare ground (Smith & Couper 1986, Smith 1987, 1993, Convey & Smith 2006, Hughes et al. 2006).
Amendment methodologies have also been attempted in the Antarctic, to date limited to the use of lights to alter exposure of natural habitats to the UV spectrum (Montiel et al. 1999, George et al. 2001), and analogous experiments have been completed in laboratory controlled environment cabinets (Rozema et al. 2001).
At non-Antarctic locations augmentation studies have also addressed CO2 enrichment (Henry 1997) and ground heating using buried heaters. Such methodologies carry severe practical constraints in terms of power and maintenance or manpower requirements, and have yet to be applied in remote and severe environments such as the Antarctic.
However, the long-term application of field manipulation methodologies, while certainly an imperfect mimic of climate model predictions, is the only practicable experimental approach to the study of biological responses to change in the field, whether in remote locations such as the Antarctic, or more familiar areas at lower latitude.
Many manipulation studies have explicitly focused on the consequences of warming or irradiation receipt, and have justifiably attracted methodological criticism through failing to take into account adequately that most actually alter not only the target but also a suite of inter-related environmental variables (Kennedy 195a, 1995b).
It is now clear that an holistic and inevitably more complex approach is required, incorporating multivariate manipulations and more complex statistical analyses (Day et al. 1999, 2001, Convey et al. 2002).
From such studies, where temperature, water and radiation regimes can be manipulated together and responses quantified in the contexts of plant biochemistry, morphology, life history and ecology, the decomposition cycle and the wider food web, it has been concluded that biological responses, while present, are often likely to be subtle and hard to detect.
While this conclusion is not simple and clear-cut, as might be desired in the non- scientific political and media realm, it does carry a message of fundamental importance. Even subtle responses involve changes in gene activity and resource allocation at cellular and organism level (Clark et al. 2004, Peck et al. 2005), and hence in life history strategy, and it is vital to understand how they may integrate through food webs and lead to considerably greater impacts at community or ecosystem levels (Day 2001, Searies et al. 2001, Convey 2003).
In addition to the indirect consequences of human activity on biological systems, acting through climate change, direct impacts are also evident.
The impacts on the Antarctic marine environment through human exploitation of marine resources may alter the relative success of different life history strategies and, through this, have impacts on both marine and terrestrial biology.
As an example of this, fishing of long-lived ground fish species in the Southern Ocean may lead to their replacement by cephalopods with much more rapid life cycles (Caddy & Rodhouse 1998).
It is not clear whether such a change is automatically reversible or whether this element of the marine ecosystem has been moved into a new stable state. Changes in the marine ecosystem may also lead to spectacular consequences for the biodiversity of terrestrial ecosystems.
Thus, the recent very rapid recovery in populations of Antarctic fur seal from near-extinction to pre-exploitation levels (Boyd 1993) has been accompanied by a large range expansion in which resting and moulting seals occupy previously non-impacted terrestrial and freshwater habitats (Hodgson & Johnston 1997, Hodgson et al. 1998), rapidly leading to destruction of these habitats and their biodiversity (Smith 1988).
Again, it is unclear whether this is a ‘temporary’ state, with the shorter fur seal life cycle allowing more rapid population recovery than is the case for competing whale species, or whether the marine ecosystem has shifted to a new state.
Impacts of climate change on marine biodiversity, biogeography, and ecosystem function
The best-known elements of Antarctica’s marine life are probably krill (Euphausla superba, a euphausiid crustacean) and their vertebrate consumers, including penguins, seals and whales.
Krill can be very abundant in some areas at sometimes, but copepods also dominate zooplankton in other areas or times. In addition to the vertebrate predators, squid (cephalopod molluscs) can be very abundant krill consumers in Antarctic waters and, in turn, are important to many higher predators.
Whilst there are many species of Antarctic copepods, pelagic fish are poorly represented. Most human focus has been, and still is, directed at these water column species, partly because of their historical importance as a resource (including the charismatic megafauna), but also more recently through their profile in the fields of tourism and conservation.
This perception of the Antarctic or Southern Ocean marine ecosystem is not entirely accurate, as the greatest contribution to the biodiversity of Antarctic waters comes from the seabed of the continental shelf (Clarke & Johnston 2003).
Here, benthic animals face scouring from the near random gouges of stranded icebergs for much of the year. Respite comes when the sea surface freezes in winter, but at higher latitudes this is accompanied by ice forming around some organisms in the shallows, encasing them and eventually, through buoyancy, ripping them off the substratum and carrying them to the surface. In the shallows, therefore, life is constantly recolonising following catastrophic disturbance.
This is also the case on larger scales of time and space - marine life has had to re-conquer the Antarctic shelf from hundreds of meters depth following each of the continent’s many glaciations (Hodgson et al. 2003).
The life that we see in the top few hundred meters depth on the Antarctic continental shelf is that which has withstood most of the last few hundred thousand years in deep (~800+m) water while being capable of colonising, living and competing at much shallower depths.
Some biologists believe this regime has promoted speciation in Antarctica and, certainly, it hosts a larger proportion of the world’s animals than would be expected given the length of coastline, or area of the continental shelf or ocean involved (Clarke & Johnston 2003).
In contrast, on land, Antarctica is a desert when compared with the Southern Ocean, in which more than 75 species representing as many as 16 phyla may be seen within just ten square meters at a single site (Barnes & Brocklngton 2003) (Fig. 6).
Traditionally, it was thought that the poles were species-poor and the tropics rich in marine biodiversity. However, although new data have roughly supported such a relationship in the Northern Hemisphere, Antarctica’s rich marine life seems to buck the trend in the Southern Hemisphere (see Arntz et al. 1994, Brey et al. 1994, Gray 2001, Barnes 2006).
Antarctic marine biodiversity is not uniformly rich, however. Some groups, such as the barnacle and true crab crustaceans, reef building corals, gastropod molluscs and reptiles are poorly represented whereas others, such as sea spiders (pycnogonans), polychaete worms, sea squirts (ascidians) and bryozoans are particularly well represented (Clarke & Johnston 2003). Even within the continental shelf area, biodiversity distribution is very uneven, with some regions proving especially rich and others impoverished (see Linse et al. (2006) for molluscs).
In the marine environment, population changes have been particularly obvious in seals and whales due to human harvesting in the last two centuries.
The population of baleen [filter—feeding) whales in the Southern Ocean has been reduced to less than 10 per cent of that reported in 1922 (Headland 1984) and Antarctic fur seals were hunted to near extinction.
The slow recovery of baleen whale populations compared with the rapid recovery of fur seal populations has been attributed to the different efficiency with which they breed and exploit their principal food source, Antarctic krill.
Despite this, it is likely that two factors will limit the continued growth of the fur seal populations: commercial krill fishing and climate change linked to ENSO events.
The former is already being monitored to determine its impact whilst the latter is apparent in data and the instrumental record (Forcada et al. 2005).
This instrumental record has shown that positive sea surface temperature anomalies at South Georgia, preceded by, and cross correlated with, frequent ENSO events between 1987 and 1998, explained extreme reductions in Antarctic fur seal pup production over a period of 20 years, again linked largely to the availability of krill.
Much scientific attention has focused on population variability in this particular euphausiid crustacean, as populations of this very important ecological (and commercial] species have varied considerably over a number of decades. Increases were thought to be related to the reduction in their predators (whales and seals), and numbers have declined in the last few decades perhaps linked either with the gradually increasing recovery of predators or the decline in winter sea-ice area (where much of the life cycle takes place) due to regional warming (Tarling & Johnson 2006).
Furthermore, Atkinson et al. (2004) found that salp (Urochordata) increases were coincident with krill decreases, and suggested this was linked to changing oceanic conditions. Understanding population changes of species in the Southern Ocean ecosystem is a complex field, as there are so many factors involved – including year to year variability in the timing, duration and nature of primary productivity and sea ice, ENSO events, human fishery intensity, and the response to/recovery from past fishing, to list a few.
Due to its long period of geographical and oceanographic isolation (though see Clarke et al. 2005, Barnes et al. 2006a), the Antarctic supports a large proportion of endemic marine species.
The level of endemism, as with biodiversity, varies between animal groups. Bryozoans are fairly typical and have levels of endemism of about 85 per cent at species level, 6 per cent at genus level and 0 per cent at family level (Barnes & de Grave 2000).
Such rates are very high compared with other areas around the world, with only small, old, oceanic islands such as those in the Hawaiian archipelago being comparable.
This does of course mean that if species are lost (become extinct) from such places they are gone forever. Both biodiversity and endemism show strong patterns around the Southern Ocean, Longhurst (1998) described Antarctic marine life as essentially consisting of one biome with two provinces — coastal and oceanic.
To the north of this an "Antarctic westerly Winds biome“ was proposed, including a higher latitude sub-Antarctic province (encompassing the sub—Antarctic islands) and a northerly province including the southern part of the Atlantic, Indian and Pacific oceans.
Studies of the benthos do not support this proposal, particularly in the emergence of the Ross Sea, the Scotia arc, the Weddell Sea, and South Georgia (amongst other areas) as separate zones (Barnes & deGrave 2000, Linse et al. 2006).
Some biogeographic patterns that became evident early in Southern Ocean research were those that followed the currents or prevailing winds, such as ‘west wind drift’. Although some marine organisms disperse as adults (those which brood their larvae), most use the larval stage as a dispersal mechanism.
The longer that larvae spend in the water column, the further, potentially, they can travel. While lecithotrophic larvae, which have their own food supply, will be limited eventually by exhaustion, planktotrophic larvae can potentially stay in the water column, assuming sufficient planktonic food supply.
Species with long lived, widely dispersing larvae have the potential to colonise new space most rapidly and are disproportionately abundant in shallow waters. However, in some groups of animals the families which brood their larvae (i.e. poor dispersers) have arguably been more evolutionarily successful (generated more species) than those with planktonic larvae although the latter have become more abundant (Poulin et al. 2002).
Furthermore, many larval forms are currently unidentifiable beyond class and sometimes even beyond phylum {Stanwell-Smith 1997), so that most of our understanding of marine ecosystems is based on studies of immature adults upwards.
This effectively leaves a black hole in knowledge relating to larval life stages, while these cineraria play significant roles in colonisation processes. Community development and ecosystem structure.
Here, sequencing approaches are now beginning to be used as a taxonomic tool. By amplifying and sequencing the same gene segment (the Cytochrome C oxidase gene [CO|]) and using it as a standardised biomarker for species it has been possible to identify specimens, define new species and to a certain extent replace traditional taxonomy.
This is called DNA brooding and enables discrimination between higher taxonomic levels across phyla and to species level in most organisms (Hebert et al. 2003a, 2003b). Although there is now an international consortium (The Barcode of Life project: http://barcoding.si.edu/), with the "simple" aim of "barcoding“ virtually every thing that lives, more applied aims are becoming apparent.
For instance, barcoding can provide a tool with which to identify and track Antarctic larvae (Webb et al. 2006) enhancing our knowledge on marine speciation, diversity, niche partitioning and many other features of ecosystems.
Human impacts on patterns in marine life within the Southern Ocean are many, although they must also be set in the context of the tremendous natural disturbances including ice (in all its forms), wind and waves and freshwater inundation.
Uncontrolled sealing and whaling led to sequential and dramatic collapses in the abundance of each target species, from the largest through to the smaller baleen whales. Following the collapse of whale stocks, Laws (1977) estimated there might be as many as 150 million tonnes of krill that the whales would have eaten now available for various seal and penguin species, and it is certainly the case that numbers of fur seals have now increased to beyond their pre-sealing numbers (Boyd 1993, Hodgson et al. 1998).
The largely uncontrolled exploitation of Southern Ocean marine resources during the Eighteenth to mid—Twentieth Centuries has led to a possibly permanent shift in marine ecosystem structure (Croxall et al. 2002), and provides an object lesson for the consequences of human mismanagement.
Even in the latter part of the Twentieth Century, and with the best efforts of the CCAMLR system, the Southern Ocean marine ecosystem continues to be the subject of a considerable illegal and unregulated fishery along with collateral ecosystem damage (bycatch) (e.g. Tuck et al. 2004), the solution to which lies within the sphere of political will rather than scientific research and advice.
To date other harvesting impacts, such as trawling on marine benthos, have been small in the Antarctic. This is largely because only very limited or scientific trawling operations have been permitted, mostly around the Heard and South Georgia archipelagos.
Elsewhere in the world trawling and demersal fishing have devastated marine communities to a level higher than even iceberg destruction (Thrush & Dayton 2002). Human use of Antarctica and adjacent regions has led to contamination of Southern Ocean fauna through accidental oil spills, and the release of sewage and garbage (see eg. Flatt 1978).
Organochlorine pesticides, PCBs and other environmentally persistent chemicals have entered the sea locally around areas of activity but also by being transported long distances to Antarctica in the air (Bargagli, 2005).
As happens elsewhere, these poisons may be concentrated both by certain organisms and as they pass up the food web, and high levels have been detected in some benthic species already (Kennicuft et al. 1995). Plastics are increasingly drifting the world’s oceans and organisms are using them as rafts.
Live benthic organisms have now been found travelling the Southern Ocean by rafting on plastics (Barnes & Fraser 2003, Barnes et al. 2006a) and attached to ship hulls (Lewis et al. 2003) in Antarctica.
Although a few marine non—indigenous species (NIS) have recently been found in the Southern Ocean (Thatje & Fuentes 2003). none are proven to be established – the only ocean for which this statement is true. Removing NIS from the land of even small islands has proved very difficult and has never been achieved from marine environments. The presence of NIS has proved to be one of the major threats to global biodiversity elsewhere in the world and will inevitably affect the Southern Ocean.
Furthermore, it is very likely that the number of vectors and rate of introduction to this region will increase, that some NIS will establish and spread, and that some of these establishers will have secondary effects and impacts on indigenous species and ecosystems. NIS are potential competitors or consumers of many indigenous species, and are now of particular concern as conditions should increasingly favour their establishment and spread, if predictions of climate models (Murphy & Mitchell 1995) and recent data (Meredith & King 2005) are true indicators of future significant Southern Ocean warming.
Increases in ultraviolet radiation reaching the Earth's surface associated with the ozone hole have impacted marine primary producers. it is well known that solar UV radiation, particularly short wavelength high energy UV—B radiation, can have a range of biological impacts, including effects on growth and reproduction, the photosynthetic system, and pigments, proteins and DNA (Harder el al. 1998).
However, scaling up what are clear results at the level of laboratory or individual species or community manipulation to the ecosystem level is a difficult and complex exercise, and there are few convincing data linking UV—B increases with changes observed at this level.
For instance, even though shifts in phytoplankton community structure have been demonstrated, considerable uncertainty remains regarding their wider impact on the ecosystem. Understanding how the Southern Ocean marine ecosystem might respond as a result of changing UVAB exposure patterns is a priority for climate scientists.
This is because many marine algae synthesise dimethylsulfonium propionate (DMSP), which is released into the ocean and subsequently atmosphere as dimethyl sulphide (DMS), a compound whose breakdown products act as cloud – condensing nuclei, leading to increased cloudiness and important impacts on the atmospheric radiation budget (Zepp et al. 2003).
High concentrations of DMS and DMSP occur in the Southern Ocean in spring as the sea ice melts, a time at which ozone depletion is at its peak. These concentration changes are partly due to increased zooplankton grazing and algal mortality, but other factors such as changes in the mixing depth and UV induced photoinhibition may also encourage the conversion of DMSP into DMS.
However, there remains great uncertainty as to the practical existence or importance of the effect of climate on this process, as the large-scale temporal and spatial variability inherent in the Southern Ocean make it difficult to identify specific UV-B effects, while some individual species have shown no changes in DMSP production during laboratory culture experiments (van Rijssel & Gleskes 2002).
The interactive effects of ozone depletion and vertical mixing on Antarctic phytoplankton have been investigated in modelling studies (Neale et al. 1998). One prediction of these studies was that exposure to UV-B radiation will strongly inhibit photosynthesis.
A 50 per cent reduction in stratospheric ozone (as a worst case scenario) was predicted to reduce daily integrated photosynthesis by up to 8.5 per cent. It seems reasonable, therefore, to be able to link increased exposure to UV-B with decreased productivity and, hence, reduced carbon draw down into the ocean.
However, the same study also notes that inhibition associated with realistic environmental variability can have a stronger influence on integrated water column photosynthesis than UV-B effects, concluding that ozone depletion can inhibit primal productivity in open waters of the Antarctic, but that natural (oceanographic and meteorological) variability in the exposure of phytoplankton to UV-B has a major role in either enhancing or diminishing its impact.
Nevertheless, it is clear that UV-B is a significant environmental stressor in the Southern Ocean ecosystem, and that its effects are enhanced by ozone depletion.
Impacts of climate change on terrestrial biodiversity and biogeography
As a foundation to assessing the significance of climate change and other anthropogenic processes on regional terrestrial biodiversity and biogeography in the Antarctic, it is appropriate to consider briefly the natural processes of colonisation and establishment in this isolated region.
Wind is a major vector for the colonisation of isolated environments, particularly for smaller organisms and/or their propagules (plants, insects, microbes), and has been strongly implicated in underlying the distribution of vegetation on the sub-Antarctic and other Southern Ocean Islands (Preest 1964, Munoz et al. 2004).
Wind has also been suggested as a major vector for freshwater Crustacea (Falla 1960, Laybourn-Parry & Marchant 1992), which have resting stages in their life histories that are capable of survival under desiccated conditions as would be experienced during aerial transfer.
For other groups, including some terrestrial arthropods and molluscs, (long distance) air dispersal is considered unimportant (Pugh & Scott 2002, Pugh 2003). Aerobiological sampling and ice core analyses have demonstrated that many terrestrial, freshwater and even marine propagules arrive above the Antarctic continent (Smith 1991, Marshall 1996), and some may be carried well inland (Kellogg & Kellogg 1996).
As yet, few data are available to allow confirmation of the proportion of such propagules that are viable, while typical meteorological conditions around the continent only rarely permit direct north-south transport, which would minimise transfer times and presumably maximise survival (Gressitt 1964, Marshall 1996). However, it is clear that. while infrequent, such transfer opportunities do occur in nature (Marshall 1996, Greenslade et al. 1999, Convey 2005).
Transport at the water surface may also be a viable route for certain groups of Antarctic terrestrial arthropods. Some, such as ameronothroid mites, include terrestrial, intertidal and marine Antarctic representatives, and show very wide ecophysiological tolerances (Marshall & Convey 2004), including the ability to survive long periods of immersion in both fresh and seawater, or periods spent on the surface or on floating debris {see also Coulson et al. 2002).
Birds are often suggested to be important transport vectors for terrestrial or freshwater species. However, there are few clear or recent demonstrations of this in action in the Antarctic, and those that exist are limited to a tow algae and microbiota (Gressitt 1964, Schlichting et al. 1978). The most plausible vector species are those that migrate to other continents, such as species of skua, gull and sheathbill (Barnes et al. 2006a).
There are few explicit examples of animals being transported into the Antarctic using other animals as vectors (zoochory; see Pugh (1997) for review), although viable terrestrial mites have been found in the guts of seals (Pugh 1994) and having passed through the guts of sub-Antarctic ducks (E Martin, pers. comm).
Plants may also be carried considerable distances as seeds in vertebrate guts, and it seems likely that some non—indigenous plants species used this route (i.e. within the guts of introduced vertebrates) in becoming established on sub-Antarctic islands (Frenot et al. 2005).
In most cases, even when propagules arrive in the Antarctic in a viable state, subsequent successful establishment remains unlikely However, given the clear demonstrations (above) through experimental climate manipulations that conditions more humid or even a degree warmer than currently experienced are sufficient to spectacularly enhance propagule growth prospects (Fig. 5b; Smith 1990,WynneWilliams 1996b), it is likely that contemporary climate change processes will enhance the likelihood of successful natural transfer and establishment of both non-indigenous and native Antarctic biota.
Until the last two to three centuries, and largely up to recent decades. Antarctica has been protected by its geographical isolation and physically extreme climate from the consequences of human activity that are felt all too keenly elsewhere on the planet.
When humans arrived on other continents and islands around the planet, this initiated waves of introduction and spread of NIS, with concurrent and often dramatic decreases and extinctions of native biota (Vitousek et al. 1997, Vines 1999, Blackburn et al. 2004).
As yet Antarctica has experienced somewhat limited anthropogenic ecosystem damage, at least on land. Here, particularly on the sub-Antarctic islands, over this time period there have been examples of local extinctions, mainly relating to burrow—nesting bird species on islands subject to rodent or cat introduction, (see Frenot et al. 2005 for review).
In that some of these species are of very restricted global distribution, there remains a clear threat to both regional and global biodiversity. Finally, over the same period, sub-Antarctic terrestrial ecosystems have become increasingly disrupted through introductions of NIS, facilitated by a range of passive and active anthropogenic vectors (Fig. 7; Chown & Gaston 2000, Whinam et al. 2004, Frenot et al. 2005, Barnes et al. 2006a).
Anthropogenic introduction is thought to be the principal vector for most such species (Chow/n et al. 1998, Frenof et al. 2005; but not for mites, see Pugh 1994), with the frequency of anthropogenic introduction and establishment outweighing that from natural processes by one to two orders of magnitude or more (Gaston et al. 2003, Gremmen & Smith 2004, Pugh 2004).
Approaching 200 terrestrial NIS are known to have established in the Antarctic region, the majority being vascular plants and insects (Frenot et al. 2005). Only two significant sub-Antarctic islands remain free from introduced vascular plants, while introductions account for over 50 per cent of angiosperm biodiversity on the major islands of South Georgia and Kerguelen, and up to 70 per cent on the cool temperate oceanic Gough Island in the South Atlantic Ocean.
In most cases, introduced plants and invertebrates have not yet spread significantly or had more than very local impacts on native communities. However, in a small number of cases these species have become aggressive invaders, displacing and/or destroying native species or communities and presenting an increasing problem in terms of ecosystem management (Frenot et al. 2005).
In contrast, most introduced vertebrates (both grazers and predators) have already had considerable impacts on native ecosystems, modifying habitat and community structure and in some cases leading to local extinction of indigenous species.
While some introductions are potentially reversible through eradication (e.g. large grazing mammals and predatory cats), others present far larger challenges (particularly rodents and many plants), while in either case it is far from clear whether these ecosystems will then recover from such perturbation to their "natural” state.
Much of this subsection has focused on the probability and direct consequences of anthropogenic introduction of NIS into the Antarctic, and the consequences this may have for local and regional biodiversity and ecosystem processes.
However, these direct consequences of human activity in the Antarctic region are inextricably linked with the indirect effects being experienced as a result of anthropogenic climate change processes.
Thus, in locations experiencing climate "amelioration" (generally understood as warming to more favourable temperatures and/or improving availability of water), as well as impacts on the native biota, parallel consequences include reduction of the previously Intense barriers to long distance natural colonisation of the Antarctic (increasing probability of successful transfer), and reduction in ecophysiological stress experienced on arrival at an Antarctic site by either natural or assisted means (increasing the probability of successful establishment) (Frenot et al. 2005).
Given the current factor by which human—assisted introduction outweighs successful natural dispersal to the Antarctic, these direct and indirect consequences of human activity are likely to act synergistically to accelerate the rate at which Antarctic ecosystems are “invaded“ by non-Indigenous species.
Individual physiology in the response to climate change
Poikilothermic animals living in the sea around Antarctica are thought typically to have poor abilities to cope with rising temperatures (Somero & De Vries 1967, Peck & Conway 2000).
In experiments most die when temperatures are raised to between 5°C and 10°C. The reason they fail at elevated temperatures IS a lack of capacity to supply oxygen to tissues (Portner 2001, Peck 2005a), and the transfer of tissues to anaerobic metabolism has been used to identify critical temperatures that delimit the long-term physiological limits for survival (Portner et al. 1999, Peck et al. 2002).
These studies tell us much about the physiological tolerances of Antarctic marine species, but these will not be the proximate causes of loss of populations or species in a changing environment. Failure here will be dictated by ecological factors (Clarke 1996, Clarke et al. in press) including competition, resource supply and reproductive life history traits.
Recent studies have, therefore, focused on animal activities, as a measure of their competence to function in a more ecological context. Data here have shown Antarctic marine species to be very temperature sensitive (Fig. 8).
Fifty per cent of specimens of the clam Laterule elliptlca lose the ability to rebury in sediment when temperatures are raised to between +2oC and +3°C (compared with typical natural ambient temperatures of -1oC to +1oC.
The limpet Nacella concinna has a similar response to rising temperature in its ability to right itself when turned over, and no specimens of the scallop Adamussium colbecki can swim when temperatures are raised experimentally to +2°C (Peck et al. 2004).
However, this loss of function is merely a gross manifestation of the massive internal biochemical and genetic changes that are occurring and in many ways represents a final indicator of stress.
Clearly these marine organisms are being affected much earlier, at the genetic level, than is revealed by physiological observation alone. Using gene chips it is possible to survey these organisms by simultaneously examining the expression of a large suite of genes and how their expression levels vary with temperature challenge, and several gene chips for Antarctic marine organisms are currently being produced (Fig. 9).
Although no Antarctic organisms have been sequenced, available DNA sequence banks contain more than100 Gigabases of sequenced DNA from over 165,000 different organisms (press release from NIH on August 22nd 2005), so that DNA studies are now possible in any non-model organism (Gracey et al. 2001, Podrabsky & Somero 2004).
Not only is it possible to survey an animal’s response to increased temperature, but also to monitor side effects in areas such as immunocompetence and fertility, which in many ways are equally important to an organism’s long—term survival if compromised by temperature.
Additionally, such methodologies can be used to evaluate the response at individual level, as not all organisms respond identically even within the same species (Oleksiak et al. 2002).
These high sensitivities of gene activity to raised temperature are likely to be good measures of a species’ ability to cope with the proximate ecological stresses in a changing environment, and will indicate those Antarctic marine species that will suffer with small elevations of ambient temperatures.
The ability to do this type of work in Antarctic organisms will enable the identification of genetic biomarkers for temperature stress, which may then be used in other more temperate species.
Antarctic organisms are particularly useful as sentinels of environmental climate change as the clean environment of the Antarctic ensures that only temperature changes are being measured and not the additional effects of pollution (present in the rest of the world).
However, taking a wider view, three lines of evidence indicate that the ability of Antarctic marine biota to withstand raised or relatively rapid changes of temperatures is more complex than these studies suggest.
First, Antarctic marine life present today has experienced a number of changes of sea temperature over its evolutionary history, on timescales between millions and tens of thousands of years (Clarke & Crame 1989).
Second, while the shallow water marine biota found around the sub-Antarctic island of South Georgia seems typically Antarctic in character (Barnes et al. 2006b}, sea temperatures around this island can often be 2°C or more higher than those at more southern Antarctic localities (Barnes et al. 2006c).
Third, marine macro—algae (Wienke &Dieck 1989) and various invertebrates (Barnes et al. 1996) present in the intertidal zone can withstand at least short—term exposure to temperatures at low tide of +10oC or more.
This final point indicates how rapidly scales of environmental variability can change with progression over the few meters separating the extreme thermal stability of Antarctic marine subtidal waters and the much greater variability of the intertidal zone (Peck et al. 2006).
Furthermore, new studies of Antarctic intertidal life have revealed many more marine species as long-term inhabitants than were previously thought to occur, that these are not specialists to this zone and that all are clearly capable of withstanding high temperatures at low water (Waller et al. 2006).
Thus, to develop a clearer picture of how Antarctic animals are likely to respond to the rising temperatures in the Antarctic region, we need to test a wider range of species – both in terms of relatedness and in their ecological or functional roles.
Whatever the true level of ecophysiological flexibility of Antarctic marine species, the data available to date suggest that terrestrial species are much more flexible. Their natural habitat fluctuates markedly in temperature, often in excess of 20°C in a single day (Peck et al. 2006), contrasting with the stability of the marine environment (Fig. 10).
Because of this, terrestrial and freshwater invertebrates have wide temperature tolerances, with many species capable of surviving well in excess of a 50“C annual temperature range (Convey 1996b, Peck 2004).
The problems from future climate change facing Antarctic marine and terrestrial species therefore vary markedly. As noted above, mean annual air temperatures on the Antarctic Peninsula have risen by 2°C to 3°C in the last 50 years.
This level of physical change in temperature presents little problem for most Antarctic terrestrial animals, because the range of temperatures experienced in their natural habitats is already wide and encompasses this increase.
This is not to say that responses to this level of change will not be expected or seen. Indeed, the warming has caused ice on the Peninsula to recede, resulting in an increase in the area available for species to colonise, an extension of the period available for activity, an increase in the energy available within this period, and an overall increase in population size for many species.
Rather, the primary problem they are likely to face comes from the amelioration of conditions to the point where alien (non—indigenous) species become capable of survival and establishment. Sea temperatures in the region west of the Antarctic Peninsula have risen by one degree in the last 50 years.
Although this is a small rise compared with that seen in air temperatures, it poses a markedly different problem for the marine species in the region. Their sensitivity to temperature rise may mean that a change of this magnitude threatens their ability to survive. It the current warming trend in sea temperatures continues, species will face thermal conditions that threaten survival in as little as the next 50-100 years.
Marine species have further problems, because they are generally long lived and often exhibit deferred maturation (Peck 2005b). They also tend to produce fewer but larger eggs than species elsewhere {Clarke 1993, Gambia et al. 2001).
These temporally restricting factors mean that their ability to adapt to changing conditions is less than those of lower latitude species. Their ability to migrate away from worsening conditions is also poor, because Antarctica’s coastline covers very few degrees of latitude, and most of the continent’s coast is the same distance from the Pole.
Thus, while animals can migrate latitudinally along long coastlines in Africa, Asia, the Americas and Australia, this is not possible for Antarctic marine species. On all major counts, therefore, Antarctic marine species are poorer at responding to change than most similar faunas elsewhere.
Discussion and Conclusions
Integrating findings across a range of biological disciplines, as illustrated in this review, helps to clarify the warning role that Antarctica and its biology can play in advancing understanding of responses to contemporary environmental change, and in providing fundamental biological lessons applicable to the rest of the planet.
Contrasting with much of the planet, Antarctic terrestrial and marine habitats have historically been buffered from sources of both natural and assisted external colonisation by their isolation and lack of human contact (Frenot et al. 2005, Barnes et al. 2006a).
Human influence in the region is now rapidly breaking these barriers down, both indirectly through climate amelioration lowering the barriers required for successful long-distance natural colonisation and establishment, and directly through the deliberate (now discontinued) and continuing accidental import of non—indigenous organisms.
The synergy between these two routes of establishment will only serve to accelerate the destruction of this protective barrier. Biological invasions obviously carry clear and important implications at a regional (Antarctic) scale, relating to biogeography and conservation.
However, in providing clear models to improve understanding of the processes of colonisation, establishment and range change, they also enhance our ability at a global scale to understand and start to mitigate one of the most insidious consequences of the recent trend of globalisation of human transport and economic activity.
While ecosystems, continents and even entire biomes are often considered in isolation, it is clear that links and teleconnections exist across planetary scales.
With an Antarctic focus, this is clear for example in the repeated identification of ENSO events in analyses of the patterns of environmental and biological variables in Antarctic studies.
It is also clear that a better understanding of processes occurring within the Antarctic, but with global implications, is urgently required. This is particularly well illustrated by the ongoing debate about the role of carbon drawdown in the Southern Ocean in the global carbon cycle, with further debate on the potential impact on this process of changes in irradiation spectrum caused through the ozone hole.
Antarctic terrestrial organisms are generally well adapted to their highly variable environment, where they often already face natural variability that far outweighs the observed and predicted levels of change.
The contrast in terms of variability with the Antarctic marine environment could hardly be more striking (Peck et al. 2006). Here, thermal stability is the norm and intense stenothermy and narrow physiological tolerances the evolutionary consequence for many biota.
There is a real risk identified that, with the combination of the levels of warming now reported at shallow depths and the lack of opportunity for migration to colder waters (other than for taxa that can migrate to greater depths), elements of the nearshore or sub-surface Antarctic marine ecosystems may be faced with conditions that they cannot tolerate or adapt to.
In contrast, some Antarctic terrestrial biota may indeed gain in the short term from aspects of contemporary climate change unless/until critical thresholds are crossed that lead to environmental stress.
The accuracy of any biological predictions we can make at local or global scales depends fundamentally on an improved level of description of the relevant environmental variables, and of the degree of flexibility available to biota in response.
Recognition is also required that impacts may be positive or negative, and consequential on either the action of one variable alone or on multiple interacting variables.
The wider lessons to be drawn from this review are multiple, and global in their implications.
To achieve any accuracy in prediction of change consequences, we require not only detailed descriptions of particular environmental trends, but also:
- a wide and integrated knowledge of their interaction(s) with other variables,
- a detailed description of natural environmental variability at scales relevant both to the small scale microhabitat and wide scale biogeographical distribution of the target biota
- a good understanding of the impacts of environmental variability and environmental trends on biogeographical distributions
- recognition of the importance, and quantification, of biological responses that are often subtle when seen at cellular or individual level, and their integration through trophic networks up to entire ecosystems
- analogous recognition of the key contribution of applying integrative approaches across biological and palaeobiological disciplines, from the molecular and cellular through to ecosystems and macroecology
Antarctic studies emphasise that a clear understanding of the consequences of climate change, even in its simpler terrestrial ecosystems, requires a considerably greater emphasis on holistic, synthetic, multidisciplinary approaches than has generally been the case to date.
While it is clear that some responses to changing environmental conditions can be very rapid and take the form of step functions, it is more generally the case that specific responses, which will often occur at the levels of genomic expression, cellular biochemistry and organismal physiology, will be subtle and hard to detect and quantify.
Nevertheless, the integration of such responses at individual level and upwards through populations to communities and ecosystems is a fundamental prerequisite of any credible attempt to model and predict the likely consequences of change elsewhere on the planet.
Acknowledgements
This paper arose from a discussion meeting held at the British Antarctic Survey on "Antarctic biology and climate change", under the auspices of the Institute (now Royal Society) of Biology.
Figure 3 is reproduced from King et al. (2003), with permission of the American Geophysical Union.
References
Arnold, RJ. & Convey, P. (1998). The life history of the diving beetle, Lancetes angusticollis (Curtis) [Coleoptera: Dytiscidae), on subantarctic South Georgia. Polar Biology 20, 158-160
Arntz, W.E., Brey, T. & Gallardo, V.A. (1994). Antarctic zoobenthos. Oceanography and Marine Bioiogy Annual Review 32, 241 -304.
Atkinson, A., Siegel, V., Pakhomov, E. &. Rothery .P. (2004). Long-term decline in krill stock and increase in salps within the Southern Ocean. Nature 432, 100-103.
Bargagli, R. (2005). Antarctic ecosystems: environmental contamination, climate change, and human impact. Ecological Studies, volume 175, Springer, Berlin.
Barnes, D.K.A. (2006). Patterns in the marine environment. In: Kaiser M, Attrill, M, Jennings, S., Thomas, D.N., Barnes, D.K.A., Brierley, A., Polunin, N., Raffaelli, D. & Le B Williams, P. (eds) Marine Ecology. Oxford University Press, Oxford.
Barnes, D.K.A. & Brockington, S. (2005). Zoobenthic biodiversity, biomass and abundance at Adelaide Island, Antarctica. Marine Ecology Progress Series 249, 145-155.
Barnes, D. K. A.& De Grave, S. (2000). Biogeography of southern polar bryozoans. Vie et Milieu 50, 261-273.
Barnes, D.K.A. & Fraser; K.P.P. (2003). Rafting by five phyla on man-made flotsam in the Southern Ocean. Marine Ecology Progress Series 262, 289-291.
Barnes, D.K.A, Hodgson, D.A., Convey, R, Allen, C., & Clarke, A. (2006a). Incursion and excursion of Antarctic biota: past, present and future. Global Ecology and Biogeography 15, 121-142.
Barnes, D.K.A., Linse, K., Waller, C., Morely, S., Enderlein, P., Fraser; K.P.P. & Brown, M (2006b). Shallow benthic fauna communities of South Georgia Island. Polar Biology 29, 223-228
Barnes, D.K.A., Fuentes, M, Clarke, A., Schloss, I.R. & Wallace, M. (2006c). Spatial and temporal variation in shallow seawater temperatures around Antarctica. Deep Sea Research II 53, 853-865.
Barnes, D.K.A., Rothery, P. & Clarke, A. (1996). Colonisation and development in encrusting communities from the Antarctic intertidal and sublittoral. Journal of Experimental Marine Biology and Ecology 196, 251-265.
Barrett, P. (2003). Cooling a continent. Nature 421, 221 -223.
Basberg, B.L. (2004). The shore whaling stations at South Georgia. Novus forlag, Oslo, 226 pp.
Bergstrom, D.M. & Chown, S.L. (1999). Life at the front: history, ecology and change on southern ocean islands. Trends in Ecology and Evolution 14, 472-476.
Bergstrom, D.M. Convey, P & Hulskes, A.H.L. (Eds) (2006). Trends in Antarctic Terrestrial and Limnetic Ecosystems. Springer, Dordrecht. (in press).
Blackburn, EM, Cassey, P., Duncan, RR, Evans, K.L. & Gaston, K.J. (2004). Avian extinction and mammalian introductions on oceanic islands. Science 305, 195571958.
Block W (1996). Cold or drought – the lesser of two evils for terrestrial arthropods? European Journal of Entomology 93, 325–339.
Block, W. & Convey, P. (2001). Seasonal and long-term variation in body water content of an Antarctic springtail - a response to climate change? Polar Biology 24, 764-770.
Boyd, I.L. (1993). Pup production and distribution of breeding Antarctic fur seals (Arctocephalus gazelle) at South Georgia. Antarctic Science 5, 17-24.
Brey, T., Klages, M., Dahm, C., Gunny, M., Gutt, J., Hain, S., Stiller, M., Arntz, W.E., Wagele, J.W. & Zimmerman, A. (1994). Antarctic benthic diversity. Nature 368, 297.
Caddy, J.F. & Radhouse, RG. (1998). Cephalopod and groundfish landings: evidence for ecological change in global fisheries? Reviews in Fish Biology and Fisheries 8, 4317444,
Chown, 5.L. & Gaston, K.J. (2000). Island hopping invaders hitch a ride with tourists in the Southern Ocean. Nature 408, 637.
Chown, S.L., Gremen, N.J.M. & Gaston, K.J. (1998). Ecological biogeography of the Southern Ocean Islands: species-area relationships, human impacts, and conservation. American Naturalist 152, 562-575.
Clark, M.S., Clarke, A., Cockell, C.S., Convey, R, Detrich III, H.W., Fraser; K.P.P., Johnston, I.A., Methe, B.A., Murray, A.E., Peck, L,S., Romisch, K. & Rogers, A.D. (2004). Antarctic genomics. Comparative and Functional Genomics 5, 230-238.
Clarke, A. (1993). Reproductive trade-offs in caridean shrimps. Functional Ecology 7, 411-419.
Clarke, A. (1996). The influence of climate change on the distribution and evolution of organisms. In: Johnston, I.A. & Bennett, A.F. (eds) Animals and temperature: phenotypic and evolutionary adaptation, Society for Experimental Biology Seminar Series, Vol. 59, pp. 375-407. Cambridge University Press, Cambridge.
Clarke, A., Barnes, D.K.A. & Hodgson, D. {2005). How isolated is Antarctica? Trends in Ecology and Evolution 20: 173.
Clarke, A. & Crame, J.A. (1989). The origin of the Southern Ocean marine fauna. in: Crame, J.A. (ed.) Origins and evolution of the Antarctic biota. Geological Society Special Publication 47, 253-268.
Clarke, A. & Johnston, MM. (2003). Antarctic marine benthic diversity. Oceanography and Marine Biology Annual Review 41, 47-114.
Clarke, A., Murphy, E,J., Meredith, M.P., King, J. C., Peck, L. S.& Barnes, D.K.A. (in press). Climate change and the marine ecosystem of the western Antarctic Peninsula. Philosophical Transactions of the Royal Society B: Biological Sciences.
Cockell, C.S., Rettberg, R,, Horneck, G., Wynn-Williams, D.D., Scherer, K. & Gugg-Helminger, A. (2002). Influence of ice and snow covers on the UV exposure of terrestrial microbial communities: dosimetric studies. Journal of Photochemistry and Photoblology B: Biology 88, 23-32.
Convey, P. (1996a). Reproduction of Antarctic Flowering plants. Antarctic Science 8, 127-134.
Convey, P. (1996b). The influence of environmental characteristics on life history attributes of Antarctic terrestrial biota. Biological Reviews 71. 191-225.
Convey, P. (1997). How are the life history strategies of Antarctic terrestrial invertebrates influenced by extreme environmental conditions? Journal of Thermal Biology 22, 429-440,
Convey, P. (2000). How does cold constrain life cycles of terrestrial plants and animals? Cryo-Letters 21, 73—82.
Convey, P. (2003). Maritime Antarctic climate change: signals from terrestrial biology. In: Domack, E., Burnett, A., Leventer, A., Convey, P., Kirby, M. & Bindschadler, R. (eds), Antarctic Peninsula Climate Variability: Historical and Palaeoenvironmental Perspectives, Antarctic Research Series, Vol. 79, American Geophysical Union, Washington, DC, pp. 145-158.
Convey, P. (2005). Recent lepidopteran records from sub-Antarctic South Georgia. Polar Biology 28, 108-110.
Convey, R (2006). Antarctic climate change and its impacts on terrestrial ecosystems. In: Bergstrom, D, Convey, P. & Huiskes, A.H.L. (eds) Trends In Antarctic Terrestrial and Limnetic Ecosystems. Springer, Dordrecht (in press).
Convey, P, Block, W. & Peat, H.J. (2003). Soil arthropods as indicators of water stress in Antarctic terrestrial habitats? Global Change Biology 9, 1718 -1730.
Convey, Fl, Pugh, P.J.A., Jackson, 0., Murray, A. W., Ruhland, CI, Xiong, ES. & Day, IA. (2002). Response of Antarctic terrestrial arthropods to multifactorial climate manipulation over a four year period. Ecology 83, 3130-3140.
Convey, R, Scott, D. & Fraser, W.R. {2003). Biophysical and habitat changes in response to climate alteration in the Arctic and Antarctic. In: Loveioy, T.E. & Hannah, L. (eds) Climate Change and Biodiversity: Synergistic Impacts, Conservation International, Center for Applied Biodiversity Science, Advances in Applied Blodioversity Science 4, 79-84.
Convey, P. & Smith, R.I.L. (2006). Responses of terrestrial Antarctic ecosystems to climate change. Plant Ecology 182, 1-10.
Convey, P & Wynn-Williams, D.D. (2002). Antarctic soil nematode response to artificial environmental manipulation. European Journal of Soil Biology 38, 255~259.
Coulson, S.J., Hodkinson, l.D., Webb, N.R. & Harrison, J.A. (2002). Survival of terrestrial soil-dwelling arthropods on and in seawater: implications tor trans—oceanic dispersal. Functional Ecology 16, 353-356.
Croxall, J.P., Trathan, R.N. & Murphy, E.J. (2002). Environmental change and Antarctic seabird populations. Science 297, 1510-1514.
Cullather, R.l., Bromwich, D.H. & van Woert, M.L. (1996). Inter—annual variations in Antarctic precipitation related to El Nino – Southern Oscillation. Journal of Geophysical Research 101,19109-19118.
Day, T.A.(2001). Multiple trophic levels in UV-B assessments - completing the ecosystem. New Phytologist 152, 1837186.
Day, TA., Ruhland, C.T. & Xiong, E (2001). influence of solar UV-B radiation on Antarctic terrestrial plants: results from a 4-year field study. Journal of Photochemistry and Photobiology B: Biology 62, 78-87.
Day, T.A., Ruhland, C.T., Grabs, C.W. & Xiong, F. (1999). Growth and reproduction of Antarctic vascular plants in response to warming and UV radiation reductions in the field. Oecologia 119,24-35.
Doran, P.T., Prisca, J.C., Berry Lyons, W, Walsh, J.E., Fountain, A.G., McKnight, D.M., Moor-head, D.L., Virginia, RA, Wall, D.H., Glow, G.D., Fritsen, C.H., McKay, C.P & Parsons, A.N. (2002). Antarctic climate cooling and terrestrial ecosystem response. Nature 415, 517-520.
Etheridge, D.M., Steele, L.P., Langenfelds, R.L., Francey, R.J., Barnola, J.-M. & Morgan, V.I. (1996). Natural and anthropogenic changes in atmospheric CO2 over the last 1000 years from air in Antarctic ice and firn. Journal of Geophysical Research 101. 4115-4128.
Falla, RA. (1960). Oceanic birds as dispersal agents. Proceedings of the Royal Society of London, Series B 152, 655-659.
Farman, J.C., Gardiner; B. G. and Shanklin, J.D. (1985). Large losses of total ozone in Antarctica reveal seasonal CIOe/Nox interaction. Nature 315, 207-210.
Forcada, J., Trathan, P.N., Reid, K. R Murphy, E.J. (2005). The effects of global climate variability in pup production of Antarctic Fur Seals. Ecology 86, 2408-2417.
Forcada, J, Trathan, EM, Reid, K., Murphy, E.J. & Croxall, J.P. (2006). Contrasting population changes in sympatric penguin species in association with climate warming. Global Change Biology 12, 1-13.
Fowbert, J.A. & Smith, R.l.L. (1994). Rapid population increase in native vascular plants in the Argentine Islands, Antarctic Peninsula. Arctic and Alpine Research 26, 290-296.
Fox, A.J. & Cooper, A.P.R. (1998). Climate-change indicators from archival aerial photography of the Antarctic Peninsula. Annals of Glaciology 27, 636-642.
Freckman, D.W & Virginia, R.A. (1997). Low-diversity Antarctic soil nematode communities: distribution and response to disturbance. Ecology 78, 363-369.
Frenot, K, Chown, S.L., Whinam, J., Selkirk, P., Convey, P., Skotnicki, M. & Bergstrom, D. (2005). Biological invasions in the Antarctic: extent, impacts and implications. Biological Reviews 80, 45-72,
Gambi, M.C., Patti, F.P., Micaletto, G. & Giangrande, A. (2001). Diversity of reproductive features in some Antarctic polynoid and sabellid polchaetes, with a description of Demonax polarsterni sp n. (Polychaeta, Sabellidae). Polar Biology 24, 883-891.
Gaston K.J., Jones A.G., Hanel, C & Chown, S.L. (2003). Rates of species introduction to a remote oceanic island. Proceedings of the Royal Society of London, series B 270, 1091-1098.
Gautier, C., He, G. and Yang, S. (1994). Role of clouds and ozone on spectral ultraviolet-B radiation and biologically active UV dose over Antarctica. in: Weiler, C. and Penhale, P. (eds) Ultravrolet radiation in Antarctica: measurement and biological effects. Antarctic Research Series, Vol. 62, American Geophysical Union, Washington, DC. pp. 83—91.
George, A.L., Murray, A. W.A. & Montiel, P,O. (2001). Tolerance of Antarctic cyanobacterial mats to enhanced UV radiation. FEMS Microbiology Ecology 37, 91-101.
Gerighausen, U., Brautigam, K., Mustafa, O. & Peter, H.-U. (2003). Expansion of vascular plants on an Antarctic island – a consequence of climate change? in Huiskes. A.H.L.
Gleskes, W.W.C., Rozema, J., Schorno, R.M.L_, van der Vies, SM. & Wolff, W.J. (eds) Antarctic Biology in a Global Context, Backhuys, Leiden, pp. 79-83.
Gracey A. K, Troll, J.V. & Samero, G.N. (2001). Hypoxia-induced gene expression profiling in the euryoxic fish Gillichthys mirabilis. Proceedings of the National Academy of Sciences 98. 1993-1998.
Gray, J.S. (2001). Marine diversity: the paradigms in patterns of species richness examined. Scientia Marina 65, 41-56
Greenslade, R, Farrow, RA. & Smith, J.M.B. (1999). Long distance migration of insects to a subantarctic island. Journal of Biogeography 26, 1161-1167.
Gremmen, N. & Smith, M (2004). The flora of Marion and Prince Edward islands. Data Analyse Ecologie, Diever, Netherlands.
Gressitt, J.L. (1964). Insects of Campbell Island. Pacific Insects Monograph 7, 1-663.
Grebe, C.W., Ruhland C.T. & Day, T.A. (1997). A new population of Colobanthus quitensis near Arthur Harbor, Antarctica: correlating recruitment with warmer summer temperatures. Arctic and Alpine Research 29. 217-221.
Hader; D.-P., Kumar, H.D., Smith, R. C. E Worrest, R. C. (1998). Effects on aquatic ecosystems. Journal of Photochemistry and Photobiology B: Biology 46, 53-68.
Harangozo, SA. (2000). A search for ENSO teleconnections in the west Antarctic Peninsula climate in austral winter. International Journal of Climatology 20, 663-679.
Headland, R.K. (1984). The Island of South Georgia.Cambridge University Press, Cambridge, 293 pp.
Hebert, P.D.N., Cywinska, A., Ball, S.L. & Dewaard, J.R. (2003a). Biological identifications through DNA barccdes. Proceedings of the Royal Society of London Series B – Biological Sciences 270, 313-321.
Hebert, P.D.N., Ratnasingham, S. & Dewaard, J.R. (2003b). Barcoding animal life: cytochrome c oxidase subunit 1 divergences among closely related species. Proceedings of the Royal Society of London Series B – Biological Sciences 270, 96-99.
Henry G.H.R. (ed.) (1997). Global Change. Biology 3 (suppl. 1).
Hodgson, D.A. & Convey, P. (2005). A 7000 year record of the oribatid mite communities on a maritime-Antarctic island: responses to climate change. Arctic, Antarctic and Alpine Research 37, 239-245.
Hodgson D.A. & Johnston N.M. (1997). Inferring seal populations from lake sediments. Nature 387, 30-31.
Hodgson D.A., Johnston N.M., Caulkett A.P & Jones V.J. (1998).Palaeolimnology of Antarctic fur seal Arctocephalus gazelle populations and implications for Antarctic management. Biological Conservation 83, 145-154.
Hodgson, D.A., McMinn, A., Kirkup, H., Cremer, H., Gore, D., Melles, M., Roberts, D. & Montiel, P. (2003). Colonization, succession, and extinction of marine floras during a glacial cycle: a case study from the Windmill Islands (east Antarctica) using biomarkers. Palaeooeanography 18, doi: 10.1029/2002PA000775.
Hodgson, D.A., Roberts, D., McMinn, A., Verleyen, E., Terry, B., Corbett, C. & Vyerrman, W. (2006). Recent rapid salinity rise in three East Antarctic lakes. Journal of Palaeolimnology, doi: 10.1007/s10933-006-9010-0
Houghton, J.T. (2001). Climate Change 2001: The Scientific Basis. Intergovernmental Panel on Climate Change, 3rd Assessment Report. Cambridge University Press, Cambridge.
Hughes, K., Ott, S., Bolter, M. & Convey, P. (2006). Colonisation processes. In: Bergstrom, D.M, Convey, P. & Huiskes, A.H.L. (Eds) Trends in Antarctic Terrestrial and Limnetic Ecosystems. Springer, Dordrecht. (in press).
Inter-governmental Panel on Climate Change (lPCC) (2001). Climate change 2001: impacts, adaptation and vulnerability. In: McCarthy, J.J., Canziani, O.F., Leary, N.A., Dokken, D.J. & White. K.S. (eds). Contribution of Working Group 2 to the Third Assessment Report of the lPCC. Cambridge University Press, Cambridge, UK.
Kellogg, D.E. & Kellogg, T.B. (1996). Diatoms in South Pole ice: Implications for eolian contamination of Sirius Group deposits. Geology 24,115,118
Kennedy, AD. (1993). Water as a limiting factor in the Antarctic terrestrial environment: a biogeographical synthesis. Arctic and Alpine Research 25. 308-315.
Kennedy, AD. (1994). Simulated climate change: a field manipulation study of polar microarthropod community response to global warming. Ecography17, 131—140.
Kennedy, A.D. (1995a). Temperature effects of passive greenhouse apparatus in high-latitude climate change experiments. Functional Ecology 9, 340-350.
Kennedy, A.D. (1995b). Simulated climate change: are passive greenhouses a valid microcosm for testing the biological effects of environmental perturbations? Global Change Biology 1, 29-42.
Kennicutt II, M.C., McDonald, S.J., Sericano, J.L., Boothe, P., Oliver. J., Safe, S., Presley, B.J., Liu, H., Wolfe, D., Wade, T.L, Crockett, A. & Bockus. D. (1995). Human contamination of the marine environment — Arthur Harbor and McMurdo Sound, Antarctica. Environmental Science and Technology 29, 1279-1287.
Kiernan, K. & McConnell, A. (2002). Glacier retreat and melt-lake expansion at Stephenson Glacier, Heard Island World Heritage Area. Polar Record 38 (207), 297—308.
King, D (2005). Climate change: the science and the policy. Journal of Applied Ecology 42, 779-783.
King, J. C. & Harangazo, S.A. (1998). Climate change in the western Antarctic Peninsula since 1945: observations and possible causes. Annals of Glaciology 27, 571-575.
King, J.C., Turner, J., Marshall, G.J., Connally, W.M. & Lachlan-Cope, T.A. (2003). Antarctic Peninsula climate variability and its causes as revealed by analysis of instrumental records. in: Domack, E., Burnett, A., Leventer, A., Convey, P., Kirby, M. & Bindschadler, R. (eds), Antarctic Peninsula Climate Variability: Historical and Palaeoenvironmental Perspectives, Antarctic Research Series, Vol. 79, American Geophysical Union, Washington. D.C. pp. 17-
Laws, R.M. (1977). Seals and whales of the Southern Ocean. Philosophical Transactions of the Royal Society of London series B 279, 81-96.
Laybourn—Parry, J. & Marchant, H.J. (1992). Daphniopsis studeri (Crustacea: Cladocera] in lakes from the Vestfold Hills, Antarctica. Polar Biology 11. 631-7635.
Lewis, P.N., Hewitt, C.L., Riddle, M. & McMinn, A. (2003). Marine introductions in the Southern Ocean: an unrecognised hazard to biodiversity. Marine Pollution Bulletin 46, 213-223.
Linse, K., Griffiths, H.J., Barnes, D.K.A. & Clarke, A. (2006). Biodiversity and biogeography of Antarctic and Sub-Antarctic Mollusca. Deep Sea Research ll 53, 985-1008.
Livermore, RA, Nankivell, A.P, Eagles, G. & Morris, P. (2005). Paleogene opening of Drake Passage (2005). Earth and Planetary Science Letters 236, 459-470.
Longhurst, SJ. (1998). Ecological geography of the sea. Academic Press. London.
Marshall, E.J. & Convey, P. (2004). Latitudinal variation in habitat specificity of ameronothrioid mites. Experimental and Applied Acarology 34. 21-735.
Marshall, WA. (1996). Biological particles over Antarctica. Nature 383, 680.
Masson, V., Vimeux, E, Jouzel, J., Morgan, V., Delmotte, M., Ciais, Ft, Hammer, C., Johnson, S., Lipenkov, V.Y., Mosley-Thompson, E., Petit, J.R., Steig, E.J., Stievenard, M., & Vaikmae,
R. (2000). Holocene climate variability in Antarctica based on 11 ice-core isotope records. Quaternary Research 54. 348-358.
McGraw, J.B. & Day, T.A. (1997). Size and characteristics of a natural seed bank in Antarctica.Arctic and Alpine Research 29, 213-216.
Meredith, MP. & King, J. C. (2005). Rapid climate change in the ocean to the west of the Antarctic Peninsula during the second half of the 20th century. Geophysical Research Letters 32, doi:10.1029/2005g102042.
Montiel, P.O., Smith, A.E. & Keiller; D.H. (1999). Photosynthetic responses of selected Antarctic plants to solar radiation in the southern maritime Antarctic. Polar Research 18, 229-285.
Mufioz, J., Felicisimc, A.M., Cabezas, F., Burgaz, A.R. & Martinez, 1. (2004). Wind as a Long-Distance Dispersal Vehicle in the Southern Hemisphere. Science 304, 1144-1147.
Murphy, J. M. & Mitchell, J.F.B. (1995). Transient response of the Hadley Centre coupled ocean—atmosphere model to increasing carbon dioxide. Journal of Climate 8, 36-514.
Neale, P.J., Davis, R.F. & Cullen, J.J. (1993). Interactive effects of ozone depletion and vertical mixing on photosynthesis of Antarctic phytoplankton. Nature 392. 585-589.
Oleksiak, M.F., Churchill, G.A. & Crawford, D.L. (2002). Variation in gene expression within and among natural populations. Nature Genetics 32, 281-266.
Peck, LS. (2004). Physiological flexibility: the key to success and survival for Antarctic fairy shrimps in highly fluctuating extreme environments. Freshwater Biology 49. 1195-1205.
Peck, LS. (2005a). Prospects for surviving climate change in Antarctic aquatic species. Frontiers in Zoology 2. doi: 10.1186/1742-9994-2-9.
Peck, LS. (2005b). Prospects for survival in the Southern Ocean: vulnerability of benthic species to temperature change. Antarctic Science 17, 497-507.
Peck, LS., Clark, M.S., Clarke, A., Cockell, C.S., Convey, P, Detrich, III, H.W., Fraser; K.P.P., Johnston, I., Methe, B., Murray, A.E., Romisch, K. &. Rogers, A. (2005).
Genomics: Applications to Antarctic Ecosystems. Polar Biology 28, 351-365.
Peck, L.S., Convey, P. 8. Barnes, D.K.A. (2006). Environmental constraints on life histories in Antarctic ecosystems: tempos, timings and predictability. Biological Reviews 81. 75-109.
Peck, LS. & Conway, L.Z. (2000). The myth of metabolic cold adaptation: oxygen consumption in stenothermal Antarctic bivalves. In: Harper, E.M. Taylor, J.D. & Creme, J.A. (eds) The Evolutionary Biology of the Bivalvia, Geological Society, London, special Publication No 177, 441-445.
Peck, L.S., Portner, H.O. & Hardewig, l. (2002). Metabolic demand, oxygen supply and critical temperatures in the Antarctic bivalve Laternula elliptica. Physiological & Biochemical Zoology 75, 1237133.
Peck, L.S., Webb, K.E. & Bailey, D.M. (2004). Extreme sensitivity of biological function to temperature in Antarctic marine species. Functional Ecology 18, 8257530.
Plait, H.M. {1978). Assessment of the macrobenthos in an Antarctic environment following recent pollution abatement. Marine Pollution Bulletin 9, 149-153.
Podrabsky, J.E. & Somero, G.N. (2004). Changes in gene expression associated with acclimation to constant temperatures and fluctuating daily temperatures in an annual killifish Austrotundulus limnaeus. Journal of Experimental Biology 207, 2237-2254.
Poole, L. & Cantrill, D.J. (2001). Fossil woods from Williams Point Beds, Livingston Island, Antarctica: a Late Cretaceous southern high latitude flora. Palaeontology 44, 1081-1112.
Portner, H.O. (2001). Climate change and temperature-dependent biogeography: oxygen limitation of thermal tolerance in animals. Naturwissenschaften 88. 625-530.
Portner, H., Hardewlg, I. & Peck, L.S.(1999). Mitochondrial correlates of critical temperature in Laternula eiliptica.Journal of Comparative Physiology 124, 179-189.
Poulin, E., Palma, A.T. & Feral, J -P (2002). Evolutionary versus ecological success in Antarctic benthic invertebrates. Trends in Ecology and Evolution 17, 218-222.
Preest, D.S. (1964). A note on the dispersal characteristics of the seeds of the New Zealand podocarps and beeches and their biogeographical significance. in: Gressitt, J.L. (ed), Pacific Basin Biogeography, pp. 415-424. Bishop Museum, Hawaii.
Pugh, P.J.A. (1994). Non—indigenous Acari of Antarctica and the sub—Antarctic islands. Zoological Journal of the Linnean Society 110, 207-217.
Pugh, P.J.A. (1997). Acarine colonisation of Antarctica and the islands of the Southern Ocean: the role of zoohoria. Polar Record 33, 113-122.
Pugh, P.J.A. (2003). Have mites (Acarina: Arachnicla) colonised Antarctica and the islands of the Southern Ocean via air currents? Polar Record 39, 239-244.
Pugh, RJ.A. (2004). Biogeography of spiders (Araneae: Arachnida) on the islands of the Southern Ocean. Journal of Natural History 38, 1461-1487.
Pugh, P.J.A. & Scott, B. (2002). Biodiversity and biogeography of non-marine Mollusca on the islands of the southern Ocean. Journal of Natural History 36, 927-952
Quayle, W.C, Convey, P., Peck, L.S., Ellis-Evans, J. C., Butler, H.G., and Peat, H.J. (2003). Ecological responses of maritime Antarctic lakes to regional climate change. in E. Domack, A. Burnett, A. Leventer, P. Convey, M, Kirby and R. Bindschadler (eds), Antarctic Peninsula Climate Variability: Historical and Palaeoenvironmental Perspectives, Antarctic Research Series, Vol. 79, American Geophysical Union, Washington. D.C. pp. 159—170.
Quayle, W.C., Peck, L.S., Peat, H., Ellis-Evans, J.C. & Harrigan, P.R. (2002). Extreme responses to climate change in Antarctic lakes. Science 295, 645. van Rijssel, M. & Gieskes, W.W.C. (2002). Temperature, light, and the dimethylsulfoniopropionate (DMSP) content of Emiliania huxleyi (Prymnesiophyceae). Journal of Sea Research 48, 1727.
Rozema, J., Broekman, H., Lud, D., Huiskes, A.H.L., Moerdijk, T, De Bakker, N., Meijkamp, B. & Van Beem, A. (2001). Consequences of depletion of stratospheric ozone tor terrestrial Antarctic ecosystems: the response of Deschampsia antarctica to enhanced UV—B radiation in a controlled environment. Plant Ecology 154, 103-115.
Sabburg, J. & Wong, J. (2000). The effect of clouds on enhancing UVB irradiance at the earth's surface: a one year study. Geophysical Research Letters 27. 3337—3340.
Schlichting, H.E., Speziale, E.J. & Zink, RM. (1978). Dispersal of algae and Protozoa by Antarctic flying birds. Antarctic Journal of the United States 13, 147—149.
Searies, P.S., Krupp, B.R., Flint, S.D. & Caldwell, M.M. (2001). Influence of solar UV—B radiation on peatland microbial communities of southern Argentina. New Phytologist 152, 213-221.
Skvarca, P. ill De Angelis, H. (2003). Impact assessment of regional climatic warming on glaciers and ice shelves of the north-western Antarctic Peninsula. In: Domack, E., Burnett, A., Leventer. A. Convey, P., Kirby, M. & Bindschadler, R. (eds), Antarctic Peninsula Climate Variability: Historical and Palaeoenvironmental Perspectives, Antarctic Research Series, Vol. 79, American Geophysical Union, Washington, D.C., pp. 69-78.
Skvarca, R, Rack, W., Rott, H. & lbarzabal y Donangelo, I (1998). Evidence of recent climatic warming on the eastern Antarctic Peninsula. Annals of Glaciology 27, 628-632.
Smith R.I.L. (1987). The bryophyte propagule bank of Antarctic fellfield soils. Symposia Biologica Hungarica 35, 233-245.
Smith R.I.L. (1983). Destruction of Antarctic terrestrial ecosystems by a rapidly increasing fur seal population. Biological Conservation 45, 55-72.
Smith, R.l.L. (1990). Signy Island as a paradigm of biological and environmental change in Antarctic terrestrial ecosystems. in: Kerry, K.R. & Hempel, G. (eds), Antarctic Ecosystems, Ecological Change and Conservation, Springer-Verlag, Berlin, pp. 32-50,
Smith, R.l.L. (1991). Exotic sporomorpha as indicators of potential immigrant colonists in Antarctica. Grana 30, 313-324.
Smith, R.M. (1993). The role of bryophyte propagule banks in primary succession: case study of an Antarctic fellfield soil. in: Miles, J. & Walton, D,W,H, (eds) Primary Succession on Land, Blackwell, Oxford, pp. 55—78.
Smith, R.l.L. (1994). Vascular plants as indicators of regional warming in Antarctica. Oecologia 99, 322-328.
Smith, R.l.L. (2001). Plant colonization response to climate change in the Antarctic. Folia Fac. Sci. Nat. Univ. Masarykianae Brunensis. Geographia 25, l9-33.
Smith, R.l.L. & Coupar, A.M. (1986). The colonization potential of bryophyte propagules in Antarctic fellfield soils. CNFRA 58. l89-204.
Somero, G.N. & DeVries, A.L. (1967). Temperature tolerance of some Antarctic fishes. Science 156, 257-258.
Somme, L. (1995). Invertebrates in Hot and Cold Arid Environments. Springer—Verlag, Berlin.
Stanwell-Smith, D. (1997). Larval ecology of benthic marine invertebrates at Signy Island, Antarctica. PhD Thesis, Open University. Milton Keynes.
Stephens, B.B. & Keeling, R.E. (2000). The influence of Antarctic sea ice on glacial-interglacial CO2 variations. Nature 404, 171-174.
Strathdee, A.T, Bale, J.S., Block, W.C., Coulson, E.J., Hodkinson, l.D. & Webb, M.R. (1993). Effects of temperature elevation on a field population of Acyrthosiphon svalbardicum (Heroiptera: Aphididae) on Spitsbergen. Oecologia 96, 457-465.
Tarling, G.A. & Johnson, M.L. (2006). Satiation gives krill that sinking feeling. Current Biology 16(3), R83-R84.
Thane, S. & Fuentes, V. (2003). First record of anomuran and brachyuran larvae (Crustaceae Decapoda) from Antarctic waters. Polar Biology 26, 279-282.
Thompson, D.W.J. & Solomon, S. (2002). Interpretation of recent southern hemisphere climate change. Science 296, 895-599.
Thrush, S.F. & Dayton, R.K. (2002). Disturbance to marine benthic habitats by trawling and dredging: Implications for Marine Biodiversity. Annual Review of Ecology and Systematics 33, 449-473.
Tripati, A., Backman, J., Henry Elderficld, H & Patrizia Ferretti, P. (2005). Eocene bipolar glaciation associated with global carbon cycle changes. Nature 438, 341-346.
Tuck, G.N., Polacheck, I, Croxall, J.P., Weimerskirch, H., Ryan, P.G.., Nel, D.E., Wayte, S. & Bulman, C.M. (2004). Modelling the impact of fishery incidental mortality on three populations of wandering albatross. In: Tuck. G.N. (ed), A comprehensive study of the ecological impacts of the world-wide pelagic Iongline Industry: Southern Hemisphere studies, CSIRO Marine Research. Hobart. pp. 149-223.
Turner, J., Colwell, S.R. & Harangozo, S. (1997). Variability of precipitation over the coastal western Antarctic Peninsula from synoptic observations. Journal of Geophysical Research 102, l3999-14007.
Turner, J., Colwell, S.R, Marshall, G.J., Lachlan-Cope, T.A., Carleton, A.M., Jones, P.D., Lagun, V, Reid, P.A. & Lagovkina, S. (2005a). Antarctic climate change during the last 50 years. International Journal of Climatology 25, 279-294.
Turner; J., Lachlan-Cope, T.A. Colwell, S.R. & Marshall, G.J. (2005b). A positive trend in western Antarctic Peninsula precipitation over the last 50 years reflecting regional and Antarctic-wide atmospheric circulation changes. Annals of Glaciology 41, 85-91.
Vaughan, D.G. (2006). Recent trends in melting conditions on the Antarctic Peninsula and their implications for ice-sheet mass balance. Arctic, Antarctic and Alpine Research 38, 147-152.
Vaughan, D.G., Marshall, G.J., Connolley, W.M., Parkinson, C.L., Mulvaney, H., Hodgson, D.A., King, J.C., Pudsey, C.J. & Turner, J. (2003). Recent rapid regional climate warming on the Antarctic Peninsula. Climatic Change 60, 243-274.
Vines, G. (1999). Mass extinctions. New Scientist Supplement “Inside Science" 126, 1-4.
Vitousek, P.M, D’Antanio, C.M., Loops, L.L., Rejmanek, M. & Westbrooks, R. (1997). Introduced species: a significant component of human caused global change. New Zealand Journal of Ecology 21, 1-16.
Waller, C., Barnes, D.K.A. & Convey, P. (2006). Ecological constraints across an Antarctic land-sea interface.Austral Ecology 31, 656-666.
Weaver; A.J., Eby, M., Fanning, A. & Wiebe, EC. (1998). Simulated influence of carbon dioxide, orbital forcing and ice sheets on the climate of the Last Glacial Maximum. Nature 261, 17-20.
Webb, K.E., Barnes, D.K.A., Clark, M.S. & Bowden, D.A. (2006). DNA barcoding: a molecular tool to identify Antarctic marine larvae. Deep Sea Research 1153, 1053-1060.
Whinam J., Chllcott N. & Bergstrom D.M. (2004). Subantarctic hitchhikers: expeditioners as vectors for the introduction of alien organisms. Biological Conservation 121, 207-219,
Wiencke, C. & Dieck, Y. (1989). Temperature requirements for growth and temperature tolerance of macroalgae endemic to the Antarctic region. Marine Ecology Progress Series 54,189-197.
Williams, M., Dunkerley, D., DeDeckker, P, Kershaw, P. & Chappell, J. (1998). Quaternary Environments. Hodder Arnold, 352pp.
Wynn-Williams, D.D (1996a). Response of pioneer soil microalgal colonists to environmental change in Antarctica. Microbial Ecology 31, 177-188.
Wynn-Williams, D.D. (1996b). Antarctic microbial diversity: the basis of polar ecosystem processes. Biodiversity and Conservation 5, 127l-1293.
Zepp, H.E., Callaghan, T.V. & Erickson, D.J. (2003). Interactive Effects of Ozone Depletion and Climate Change on Biogeochemical Cycles. Photochem. Photobiol. Sci. 2, 51-61 2003.
This paper was initially published in 2007
Download pdfFigures
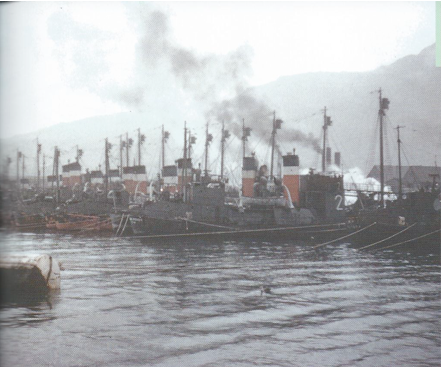
Figure 1. Whaling vessels moored at a shore-based whaling station on South Georgia, at the height of the shore based whaling industry during the first half of the Twentieth Century. (photo: N.A.G. Leppard, British Antarctic Survey Image Collection)
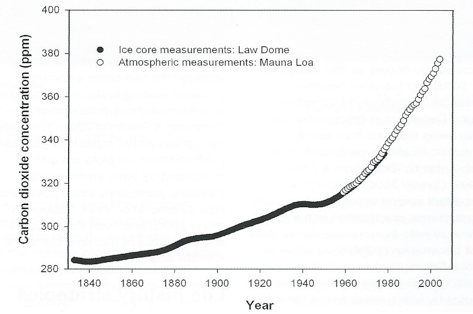
Figure 2. Historical concentrations of atmospheric carbon dioxide over the last 160 years, inferred from ice core data from the Law Dome ice core (Etheridge et al. 1996). Direct atmospheric measurements from the Manual Loa record (World Data Center for Atmospheric Trace Gases) over the last century are superimposed.
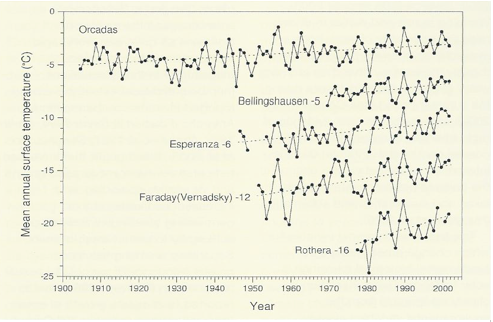
Figure 3. Annual mean surface air temperature trends at selected stations along the Antarctic Peninsula and Scotia arc, from Orcadas Station (Laurie Island. South Orkney Islands, c.60oS to Rothera Station (Adelaide Island, c. 68oS). Records other than for Orcados have been offset by the amounts shown for clarity (from King et al. 2003)
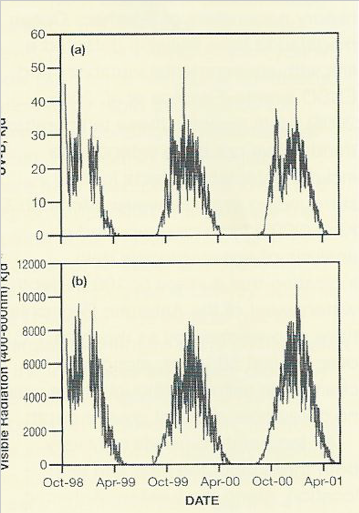
Figure 4. Change in the intensity of shorter wavelength UV—B radiation received at ground level at Rothera Research Station (Adelaide Island) as a result of spring ozone hole formation. Note that peak UV-B levels early in the austral spring are enhanced and reach levels comparable with midsummer (panel a), while no such increase is seen in levels of visible radiation (panel b) or UV-A radiation (not shown) (figure modified from Convey 2005).
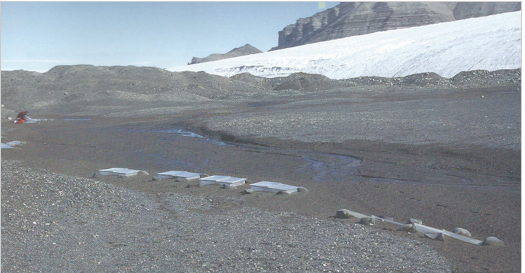
Figure 5a. An example of the deployment of passive cloches as part of a long-term study of the effects of environmental conditions and variation on the microbial, plant and invertebrate communities of soils at a site on southern Alexander Island (72°S) near the southern boundary of the maritime Antarctic (see Convey & Wynne-Williams 2002).
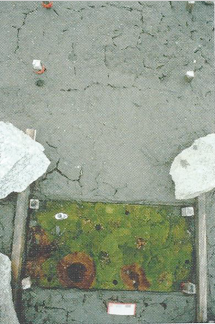
Figure 5b. An illustration of the rapid development of a native bryophyte community from the soil propagule bank on ground bare to the naked eye, as a result of placement of a cloche at Jane Col, Signy Island (60"S) (see Smith 1990, 2001). Photos: R. I. L. Smith/BASE.
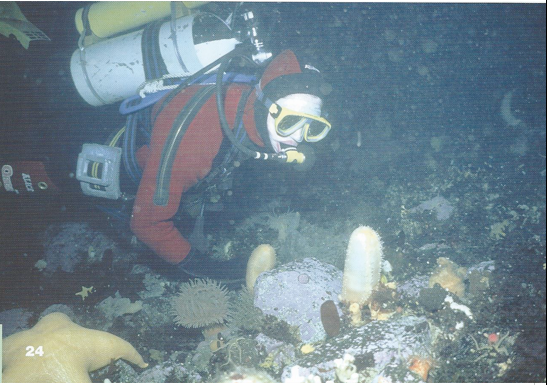
Figure 6a. Benthic diversity and biomass is patchily high on the Antarctic continental shelf (marine environment). A diver examines the benthic Community at 25 m depth during a winter dive under sea ice near Rothera Research Station. Photo: S. Brockington (BAS).
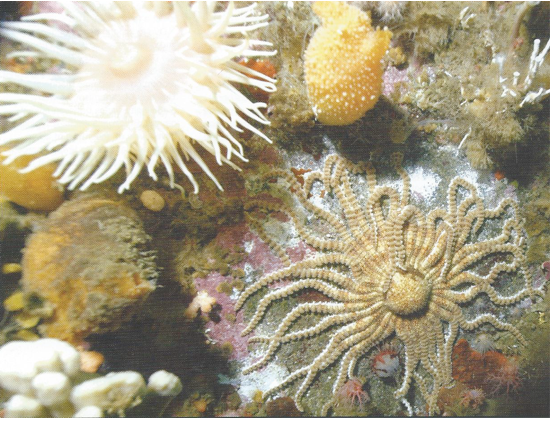
Figure 6b. Diverse encrusting Community at 20 m depth near Rothera Research Station Photo: D. Smale/BAS
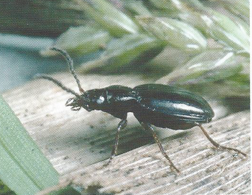
Figure 7a. The predatory carabid beetle Trechisibus antarcticus, introduced to South Georgia, an island with no native insect predators.
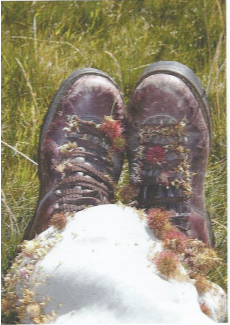
Figure 7b. Seeds of the flowering plant Acaena mageiianica attached to clothing, illustrating the potential for human activity to inadvertently transfer viable propagules between locations.
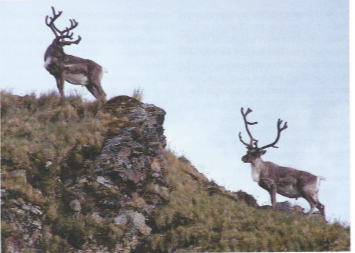
Figure 7c. Reindeer were introduced to South Georgia to provide food and recreation for whaling station staff in the early Twentieth Century, and their grazing activity has led to considerable restructuring of plant communities; here seen feeding on a sward of the introduced grass Poa annua, Whose rapid spread on the island is also facilitated by the movement of reindeer:
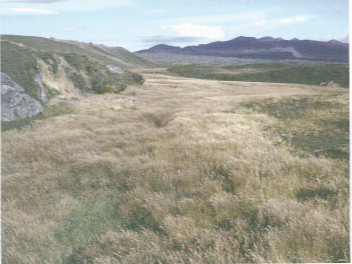
Figure 7d. Extensive sward of the introduced grass Agrostis stoiom'fera on Marion island, where it has locally almost completely displaced native vegetation
Figure 7 photos: BAS, P. Rodhouse/BAS, N. Gremmen
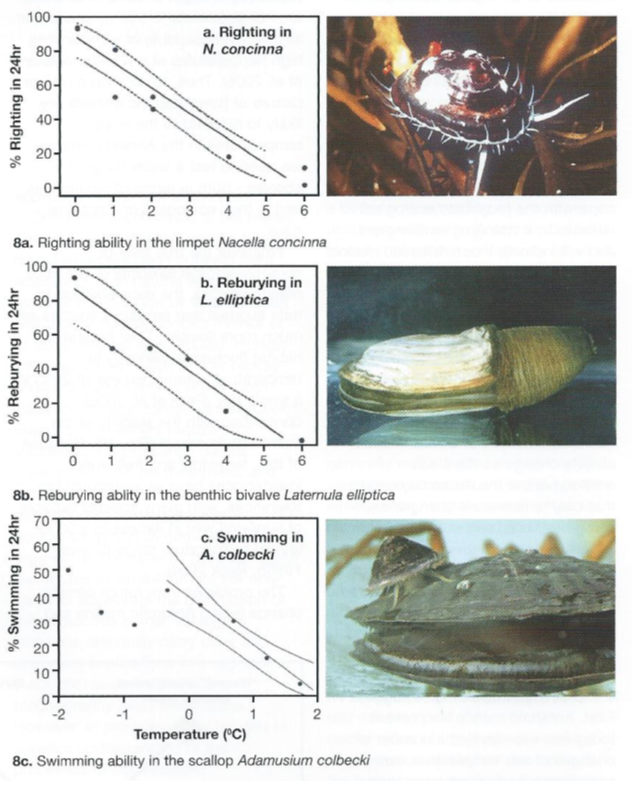
Figure 8. Illustration of the tight stenothermy that is typical of many Antarctic marine Invertebrates, and leads to a rapid failure to complete routine biological functions with even a small increase in temperature (figures modified from Peck et al. 2004)
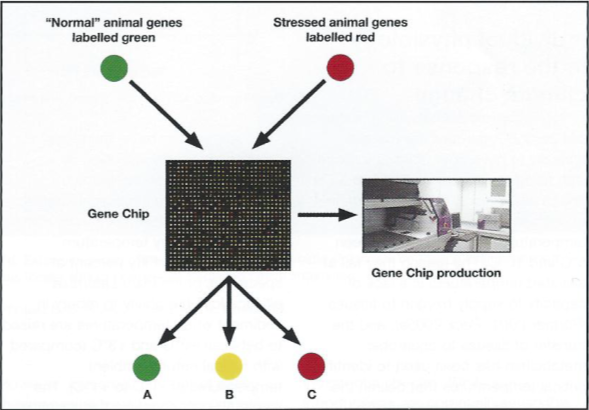
Figure 9. How a gene chip works. The genes from a "normal" animal are labelled with a fluorescent green tag and the genes from a "treated" animal are labelled with a fluorescent red tag. The "treatment“ can represent different environmental conditions such as warmer seas, drier habitat etc, These can be from animals taken directly from the field or resulting from experiments in the controlled environment of the laboratory. The green and red labelled genes are mixed and incubated with a gene chip. if one of the labelled genes matches a spot of DNA on the chip, it sticks. The chip is washed and then scanned with a laser, which makes the green and red gene tags fluoresce. If a spot looks green, then this means that the gene is more active in the normal animal. if a spot looks red. then that gene is more active in the "treated“ animal, and finally it a spot is yellow. then the gene is equally active in both sets of animals. This means that we can work out which genes are more active under particular environmental conditions and determine if the animal is adapting or "coping" with environmental change.
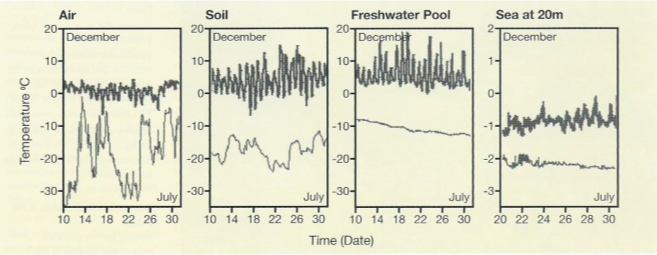
Figure 10. Different scales of environmental temperature variation in summer (December) and winter (July) in Antarctic terrestrial. freshwater and marine environments; note particularly the much reduced temperature scale in the latter